Bioimpacts. 12(2):155-169.
doi: 10.34172/bi.2022.23900
Review
Recent advancements in cell-based models for auditory disorders
Jake Langlie 1, #
, Ariel Finberg 1, #, Nathalie B. Bencie 1, Jeenu Mittal 1, Hossein Omidian 2, Yadollah Omidi 2
, Rahul Mittal 1, Adrien A. Eshraghi 1, 3, 4, 5, * 
Author information:
1Department of Otolaryngology, Cochlear Implant and Hearing Research Laboratory, University of Miami Miller School of Medicine, Miami, Florida, USA
2Department of Pharmaceutical Sciences, College of Pharmacy, Nova Southeastern University, Fort Lauderdale, FL 33328, USA
3Department of Neurological Surgery, University of Miami Miller School of Medicine, Miami, Florida, USA
4Department of Biomedical Engineering, University of Miami, Coral Gables, Florida, USA
5Department of Pediatrics, University of Miami Miller School of Medicine, Miami, Florida, USA
#Contributed equally to this work.
Abstract
Introduction:
Cell-based models play an important role in understanding the pathophysiology and etiology of auditory disorders. For the auditory system, models have primarily focused on restoring inner and outer hair cells. However, they have largely underrepresented the surrounding structures and cells that support the function of the hair cells.
Methods:
In this article, we will review recent advancements in the evolution of cell-based models of auditory disorders in their progression towards three dimensional (3D) models and organoids that more closely mimic the pathophysiology in vivo.
Results:
With the elucidation of the molecular targets and transcription factors required to generate diverse cell lines of the components of inner ear, research is starting to progress from two dimensional (2D) models to a greater 3D approach. Of note, the 3D models of the inner ear, including organoids, are relatively new and emerging in the field. As 3D models of the inner ear continue to evolve in complexity, their role in modeling disease will grow as they bridge the gap between cell culture and in vivo models.
Conclusion:
Using 3D cell models to understand the etiology and molecular mechanisms underlying auditory disorders holds great potential for developing more targeted and effective novel therapeutics.
Keywords: Cell-based models, Auditory system, Spiral ganglion neurons, Organoid model, 3D model, Stem cell
Copyright and License Information
© 2022 The Author(s)
This work is published by BioImpacts as an open access article distributed under the terms of the Creative Commons Attribution License (
http://creativecommons.org/licenses/by-nc/4.0/). Non-commercial uses of the work are permitted, provided the original work is properly cited.
Introduction
Cell-based models have been vital for understanding the physiology of tissues and organs. These models have aided in comprehending the molecular pathways implicated in disease and have played an essential role in high-throughput drug screening.
1,2
In the field of otology many individual cell lines and tissues of the inner ear have been replicated with cell-based models, including both sensory and non-sensory cells.
3
In the past, cell-based models have focused on replicating inner and outer hair cells, the primary functional components of sensorineural hearing. Recently, however, the field is evolving with the modeling of non-sensory structures, including neurons and supportive cells.
4
The emerging use of induced pluripotent stem cells (iPSCs), as well as tissue-derived mesenchymal stem cells (MSCs) and embryonic stem cells (ESCs), have led to this shift and thus have been essential in modeling the inner ear.
5,6
To best model and replicate the pathophysiology of inner ear disorders, it is essential to shift from the standard two-dimensional (2D) in vitro models and transition to new 3D models such as organ-on-a-chip and organoids.
7
The advancement of 2D to three-dimensional (3D) cell cultures has produced in vitro models of the inner ear that more closely mimic pathological states. Organoid models allow for the manipulation of a single diseased cell line encompassed within a greater organ-based model, substantially increasing our understanding of the pathophysiology of auditory disorders. In this review, we aim to go beyond the modeling of sensory hair cells and take a deep dive into modeling the accessory structures of hearing, including spiral ganglion neurons and supporting cells. Collectively, this review will discuss the current state of 2D cell-based models of inner ear structures before advancing to the successes and limitations of 3D models of the inner ear.
Development and anatomy of the auditory system
The human auditory system consists of the external, middle, and inner ear which work synchronously to transmit sound to the auditory cortex of the brain. To date, models of the cochlea and surrounding structures have been vital to advance our understanding about the pathologies of the inner ear, including sensorineural hearing loss (SNHL). In this section, we will discuss the development and anatomy of the inner ear. A schematic representation of the inner ear is provided in Fig. 1.
Figure 1.
Schematic representation of the inner ear. (A)
The cochlea that converts the vibrations of the cochlear liquid and inner ear organs into neural signals. (B) Modiolus located within the shell of the cochlea are the membranes in cross-section, which house the spiral ganglion, cochlear nerve, and spiral organ.(C) The organ of Corti which is made up of sensory cells that transduce auditory signals into the action potential of nerve impulses.
Figure 1.
Schematic representation of the inner ear. (A)
The cochlea that converts the vibrations of the cochlear liquid and inner ear organs into neural signals. (B) Modiolus located within the shell of the cochlea are the membranes in cross-section, which house the spiral ganglion, cochlear nerve, and spiral organ.(C) The organ of Corti which is made up of sensory cells that transduce auditory signals into the action potential of nerve impulses.
Development of the inner ear
Four weeks after conception, the precursors of the inner ear begin to materialize from the ectoderm.
8
The inner ear structures, as well as the cochleovestibular ganglion (CVG), arise from the otic placode, a specific zone within the ectoderm. The otic placode eventually gives rise to the otic vesicle, which is composed of the primordial inner ear and the CVG.
9
Four different cell types arise from the otic placode with precise topological distribution, including the sensory neurons, supporting cells, sensory hair cells, and the secretory cells of the otic epithelium.
10
These cell types give rise to ten unique cell lines that encompass the region in and around the otic capsule.
8
Anatomy of the inner ear
The anatomy of the inner ear can be subdivided into two components, the sensorineural structures of the cochlea and the vestibular organs. In this section, we will specifically focus on the sensorineural structures of the inner ear.
Bony structures of the cochlea
The cochlea itself has a bony center known as the modiolus (Fig. 1). The modiolus is the central structure of the cochlea and all other structures are considered lateral. Surrounding the modiolus is the spiral lamina, a bony corkscrew structure that extends from the apex to the base of the cochlea.
Divisions of the cochlea
Two membranes extend along the spiral lamina that divide the cochlea into three compartments: (i) scala vestibuli, (ii) scala tympani, and (iii) scala media. The upper membrane is the basilar membrane, dividing the scala tympani from the scala media, and it supports the hair cells within the organ of Corti. The vestibular membrane, also known as Reissner’s membrane, divides the lower layers of the cochlea, the scala vestibuli, and the scala media. There are three turns of the scala as they wrap around the modiolus: the basal, middle, and apical turns. The apex, joining the scala tympani and the scala vestibuli, is also known as the helicotrema. On the basal side of the cochlea, the oval window and the round window form the functional termination of the scala vestibuli and the scala tympani, respectively.
Structural regions of the cochlea
The cochlea can be divided into two structural regions, each containing a unique extracellular fluid matrix that functions in signal conduction within the inner ear. The outer labyrinth is more commonly known as the osseous (bony) labyrinth. It is a series of canals in the temporal bone that contain perilymph, an extracellular fluid with high sodium concentration. The membranous labyrinth is suspended within the osseous labyrinth and contains endolymph with high potassium concentration. The hair cells are located in the membranous labyrinth and function in the sensory reception of hearing.
Innervation of the cochlea
From distal to proximal in reference to the central nervous system (CNS), the bipolar primary afferent neurons are the peripheral neurons that leave the hair cells of the organ of Corti. The bipolar primary afferent neurons channel into the modiolus through the habenula perforata. The cell bodies of these peripheral spiral ganglion neurons are found inside Rosenthal’s canal within the modiolus. The spiral ganglion nerve fibers merge to form the cochlear branch of cranial nerve VIII. Cranial nerve VIII (CNVIII) is more commonly referred to as the vestibulocochlear nerve. CNVIII travels to the primary auditory cortex located in the superior temporal gyrus of the temporal lobe.
11
Cells of the cochlea
There are two distinct regions of the primary hair cells of the cochlea. The inner and outer hair cells are known as types I and II, respectively. They are named based on their anatomic position with respect to the modiolus within the organ of Corti. The hair cells, functioning as mechanoreceptors, transduce mechanical signals into neurological potentials. Mechanosensing organelles (stereocilia) are found on the hair cells’ apical surface and are involved in the transmission of sound via the generation of action potentials. Furthermore, multiple cells including Hensen’s cells, Claudius cells, Deiters’ cells, inner supporting cells, and inner and outer Pillar cells play supporting roles in nutrition, signal transduction, and homeostasis in the inner ear.
12
Inner ear structures and cell-based models
Although the anatomy of the inner ear was elucidated decades ago, the ability to replicate it with 3D models is a recent and evolving technique. Most publications have focused on the generation of the hearing structures, specifically the inner hair cells, without addressing the vestibular structures of the inner ear that function in balance. Therefore, little has been accomplished for the development of a 3D and organ-on-a-chip model to study the molecular functionalities of the inner ear.
Sensory structures of the inner ear
Most cell-based models of the auditory system have focused on the regeneration of hair cells previously lost due to genetic and/or environmental causes. Cell-based models have yielded two major strategies to regenerate cells in the human ear. These include the use of exogenous cells to replace injured hair cells, neurons, and accessory cells, and the endogenous differentiation of stem cells into newly generated hair cells.
13
A multitude of in vitro studies of iPSCs of inner and outer hair cells have been generated in the hopes of new therapies and drug discoveries, as well as the elucidation of the pathophysiology of SNHL. It has been found that human fetal auditory stem cells can be expanded and differentiated in vitro to hair cell-like cells and functional auditory neurons that may assist in the development of cell-based therapies for hearing loss.
14
The cultures generated have been demonstrated to possess the critical markers of progenitor auditory cells including NESTIN, SOX2, GATA3, and PAX2.
15
A key reprogramming mechanism to generate inner ear cell lines is through the use of viruses. A study successfully reprogrammed mouse embryonic fibroblasts to become inner ear hair cells. Having capitalized on the retrovirus system, they exogenously expressed four transcription factors (Six1, Atoh1, Pou4f3, and Gfi1). It was observed that the cells resembled mouse hair cells as evident from protein expression profiles as well as the expression of hair cell biomarkers such as Myosin VIIa and Parvalbumin (Fig. 2).
16
With the generation of iPSCs from patient fibroblasts and the subsequent successful passaging of cell lines, there is hope that a reimplantation model to treat SNHL will soon be generated.
5,17,18
Figure 2.
Mouse embryonic fibroblasts overexpressing Six1, Atoh1, Pou4f3, and Gfi1 (SAPG) exhibits immunostaining for hair cell markers. (A) A diagram of a mouse's inner ear showing the vestibular system (green) and the cochlea of the auditory system (red). (B) Schematic of experimental design for transcription factor-mediated reprogramming. (C) Images of MEFs reprogrammed with Six1, Atoh1, Pou4f3, and Gfi1 fixed at 14 days post-infection (dpi). Scale bar 50 µm. (D) Quantification of Atoh1-nGFP and triple-positive cells (Atoh1-nGFP+/MyosinVIIa+/Parvalbumin+). (*P<0.05, **P<0.01, ***P<0.001, ****P<0.0001). Adapted from Menendez et al.
16
Figure 2.
Mouse embryonic fibroblasts overexpressing Six1, Atoh1, Pou4f3, and Gfi1 (SAPG) exhibits immunostaining for hair cell markers. (A) A diagram of a mouse's inner ear showing the vestibular system (green) and the cochlea of the auditory system (red). (B) Schematic of experimental design for transcription factor-mediated reprogramming. (C) Images of MEFs reprogrammed with Six1, Atoh1, Pou4f3, and Gfi1 fixed at 14 days post-infection (dpi). Scale bar 50 µm. (D) Quantification of Atoh1-nGFP and triple-positive cells (Atoh1-nGFP+/MyosinVIIa+/Parvalbumin+). (*P<0.05, **P<0.01, ***P<0.001, ****P<0.0001). Adapted from Menendez et al.
16
Non-sensory structures of the inner ear
Most research and current models of SNHL have focused on the sensory structures of the inner ear. To create a 3D model of the inner ear, however, other structural elements such as accessory cells, neurons, and extracellular components must be mimicked. This section focuses on generated models of non-sensory cells of the inner ear.
Supporting cells of the cochlea
The roles of supporting cells of the inner ear are still being elucidated, but emerging literature is showing that these cells have essential roles in maintaining the integrity of spiral ganglion neurons and hair cells. Each supporting cell line within the mammalian inner ear plays a distinct role in its specific anatomic location in the cochlea. Emerging models of supporting cells of the cochlea are reviewed in Table 1.
Table 1.
Models of non-sensory structures of the inner ear
Model
|
Findings
|
Ref.
|
Inner ear supporting cells |
• Secrete heat shock protein, HSP70, protecting sensory hair cells.
|
19
|
• Supporting columnar epithelial cells found within Kolliker’s organ provide support by spontaneously releasing ATP to the inner ear and spiral ganglion neurons.
|
20
|
Deiters’ cells |
• Anchored to the basement membrane
• Provide structural support to the outer hair cells
• Provide a surface to receive nutrients via microtubules
|
21,22
|
• Function in cell-to-cell signaling via gap junctions to allow for long-distance communication.
|
23
|
Hensen’s cells |
• Arise from a disordered, undifferentiated layer of cells at around day 14 via mechanical forces patterning hair cell development.
|
24
|
• Lateral inhibition, provided through Notch signaling, functions in the targeted differentiation of otic precursors into Hensen’s cells.
|
25
|
Pillar cells |
• Hey2 block hair cell differentiation and activated by FGF.
• Mutation of transcription factor caused Pillar cells to become sensitive to Notch signaling loss, permitting differentiation into hair cells.
|
25
|
• Express genes encoding machinery for the specialization of sensory hair cells in the basolateral, apical, and synaptic membranes of the inner ear.
|
26
|
• Function in formation of tunnel of Corti, a supporting structure located between the outer and inner hair cells.
|
27
|
Claudius cells |
• AQP4, an aquaporin channelon Hensen's and Claudius cells, may contribute to the ion and volume homeostasis.
• AQP4 facilitates osmotically driven water movements within sensory epithelium of inner ear.
|
28
|
• Ablation of connexin26, a gap junction protein, causes Claudius cell death and hearing impairment via gap junction dysfunction.
|
29
|
Inner ear supporting cells
Similar to glial cells and astrocytes of the central nervous system, supporting cells of the organ of Corti play essential roles in signaling, nutrition, and proliferation.
30
Recent studies have revealed that inner ear supporting cells function in the protection of sensory hair cells by secreting HSP70, a heat shock protein.
19
They have also been shown to provide nutritional support through releasing necessary proteins and transcription factors for the survival of the SGNs and inner hair cells.
30
Deiters’ cells
Deiters’ cells provide structural support to the outer hair cells by providing a surface for the direct absorption of nutrients.
31
Deiters’ cells, along with Hensen’s cells, function in cell-to-cell signaling via gap junctions to allow long-distance communication within their basilar formation.
23
Purified stem cell-based models of Deiters’ cells have been successfully generated. However, the models are challenged by the unknown molecular markers that allow for the proliferation of these supporting cells and whether there is an absence or blockage of regenerative signals in the differentiation pathway.
21
Hensen’s cells
Hensen’s cells are structural support cells that lie on the lateral side of the outer hair cells within the organ of Corti. These cells have been differentiated in mice and shown to arise from a primordial layer of cells in about two weeks of culture.
24
Researchers have shown that lateral inhibition, provided through Notch signaling, functions in the targeted differentiation of otic precursors into Hensen’s cells.
32,33
Pillar cells
Pillar cells provide mechanical coupling between the inner and outer hair cells and their underlying basement membrane.
26
These cells have been mimicked with the aid of biomaterials and function in the formation of the tunnel of Corti, a supporting base that is located between the outer and inner hair cells.
27
Claudius cells
Claudius cells expand from the Hensen’s cells to the epithelium of the spiral prominence where they form the outer sulcus.
28
Claudius cells are bathed in the endolymph and joined by tight junctions to prevent the endolymph from contacting the hair cells. In direct contact with the hair cells, endolymph can be cytotoxic.
29
Endolymph, perilymph, and the generation of extracellular fluid
In 3D cell-based and organoid models, the normal activity of the inner ear is dependent on the electrical potential of the endolymph and perilymph.
34,35
A study of the introduction of exogenous stem cells to the inner ear found that there are factors in the microenvironment, including the perilymph and endolymph, that control the differentiation of stem cells into cells of the inner ear such as SGNs, hair cells, and supporting cells.
36
Therefore, these extracellular fluids, along with their respective electrolyte concentrations, must be mimicked to generate a proper inner ear model capable of generating electrical potentials.
Models have elucidated that the loss of bicarbonate ions causes endolymphatic acidification, leading to deafness, and that accumulation of calcium in the membranous labyrinth can lead to the death of the inner hair cells.
37
The endolymph has been characterized as a harsh environment for cell growth, and transplantation of pluripotent stem cells into this area to treat SNHL has yielded not very promising results. However, the sodium-rich perilymph is an ideal and more favorable environment in which to inject stem cells. Nevertheless, it is uncertain how cells can transverse the tightly joined scala media to migrate to their native anatomical position.
38
The key models of the endolymph and perilymph are highlighted in Table 2.
Table 2.
Models of extracellular matrix and it’s production: endolymph, perilymph, pericytes of the stria vascularis and vestibular dark cells
Model
|
Findings
|
Ref.
|
Endolymph |
• Loss of bicarbonate ions causes deafness via endolymphatic acidification.
• Accumulation of calcium in the membranous labyrinth leads to death of inner hair cells.
|
37
|
Perilymph |
• Sodium-rich perilymph provides more favorable environment to inject stem cells compared to the endolymph.
|
38
|
Endolymph and Perilymph |
• Normal functioning of inner ear dependent on electrical potential of endolymph and perilymph.
|
4
|
• Factors in microenvironment of perilymph and endolymph control differentiation of stem cells into both neural tissues and cells of the organ of Corti.
|
36
|
Pericytes of the Stria Vascularis and Vestibular Dark Cells |
• Dark cell area and stria vascularis generate ionic regulation of the endolymph and perilymph.
• K+ diffusion potential across the apical membrane contribute to the electrical potential.
|
39
|
• Essential to generate supporting cells of the inner ear in a multi-cellular model to create a homeostatic and self-proliferative environment.
|
40
|
• Cells express the typical markers of the pericytes of the stria vascularis including PDGFRβ, α-SMA, and NG2.
• Cells also characteristically negative for endothelial marker von Willebrand factor.
|
41
|
PDGFRβ = platelet-derived growth factor receptorβ; α-SMA = α-smooth muscle actin; NG2 = neural glial antigen 2.
Pericytes of the stria vascularis and vestibular dark cells
The pericytes of the stria vascularis and the dark cell area of the vestibular organs generate the ionic regulation of the endolymph.
34
These cells are essential to generate in cell-based models to create a homeostatic and self-proliferating environment.
34
A recent bovine model was able to successfully culture the pericytes of the stria vascularis. The cultured pericytes expressed the typical markers including platelet-derived growth factor receptor β (PDGFRβ), α-smooth muscle actin (α-SMA), and neural glial antigen 2 (NG2) (Fig. 3). They were also negative for the endothelial marker von Willebrand factor.
41
This may be a crucial step in generating a human model of the stria vascularis that can be used to assess ototoxic activity and dysregulation of the ions within the perilymph and endolymph. The key models of the pericytes of the stria vascularis and vestibular dark cells are highlighted in Table 2.
Figure 3.
Characterization of isolated BCP.
Green fluorescence indicates the immunostaining for NG2 (panels a, a′, and a′′) and vWF (panels d, d′, and d′′) in HREC, BCP, and HRPC, respectively. Red fluorescence indicates immunostaining for PDGFRβ (panels b, b’, and b′′) and α-SMA (panel c, c′, and c′′) in HREC, BCP, and HRPC, respectively. Blue fluorescence depicts cell nuclei, stained by DAPI. Magnification: ×20; scale bars: 100 µm.
α-SMA = α-smooth muscle actin; BCP = bovine cochlear pericytes; DAPI = 4′,6-diamidino-2-phenylindole; HREC = human retinal endothelial cells; HRPC = human retinal pericytes; PDGFRβ = platelet-derived growth factor receptor β; vWF = Von Willebrand Factor. Reproduced with permission from Giurdanella et al.
41
Figure 3.
Characterization of isolated BCP.
Green fluorescence indicates the immunostaining for NG2 (panels a, a′, and a′′) and vWF (panels d, d′, and d′′) in HREC, BCP, and HRPC, respectively. Red fluorescence indicates immunostaining for PDGFRβ (panels b, b’, and b′′) and α-SMA (panel c, c′, and c′′) in HREC, BCP, and HRPC, respectively. Blue fluorescence depicts cell nuclei, stained by DAPI. Magnification: ×20; scale bars: 100 µm.
α-SMA = α-smooth muscle actin; BCP = bovine cochlear pericytes; DAPI = 4′,6-diamidino-2-phenylindole; HREC = human retinal endothelial cells; HRPC = human retinal pericytes; PDGFRβ = platelet-derived growth factor receptor β; vWF = Von Willebrand Factor. Reproduced with permission from Giurdanella et al.
41
Spiral ganglion neurons and inner ear innervation
The main bipolar neurons that directly innervate the hair cells are the SGNs. These cells serve as a relay station between the CNS and the hair cells.
42
The cell bodies of the SGNs are clustered within Rosenthal’s canal.
43
The loss of peripheral neural input to the auditory nerve causes sensorineural deafness, stemming from either direct loss of the sensory structures, the hair cells, or loss of conduction of the electrical signal via damage to the SGNs or cranial nerve.
44
The organ of Corti is innervated by afferent SGNs and efferent brain-stem-derived motor neurons. To mimic these structures successfully in vitro, it is essential to have nearby neural-crest-derived Schwann cells to form myelin.
8,33,45
Historically, it was believed that the spiral ganglion cells degenerated soon after the inner hair cells (IHCs) of the organ of Corti die.
46
However, a study demonstrated that the spiral ganglion neurons often have delayed degeneration and survive for months after the death of IHCs.
47
It was observed that neuron survival persists beyond the death of IHCs, suggesting that the loss of SGNs is likely due to trauma rather than direct loss of the hair cells. Therefore, the hypothesis that IHCs were the main source of survival factors for SGNs was debunked, and it was proposed that other cells, most likely supporting cells in the cochlea, are the main source of survival factors.
Unlike the previous hypothesis, SGNs do not rely solely on hair cell stimulation for survival. They have been shown to rely on neutrotrophin-3 and glial-derived neurotrophic factors from accessory and supporting cells. This was shown in an in vitro model where SGNs survived for several months after hair cell death.
48
Cell death inhibitors have also been used in animal models to preserve SGN populations after hair cell death.
48
Therefore, the longstanding dogma that sensory hair cells were necessary for the proliferation and survival of SGNs has been overturned.
Cochlear implants, innervation, and regeneration
One of the essential processes to recognize in generating cell-based models of the inner ear is the physiological death and loss of hair cells, in large part due to aging. It has been well established that the cochlear implant is the mainstay of therapy for patients suffering from severe SNHL.
49-52
Cochlear implants may have challenges in providing auditory rehabilitation if the hair cells are not the principal deficit, with the deficit instead lying within the bipolar neurons, spiral ganglion cell bodies, or cochlear branch of CNVIII.
42,44,53
Auditory neuropathy is a specific subset of deafness with relative preservation of the hair cells but damage to the SGNs.
A study analyzed the differences in hearing recovery comparing cochlear implants alone to cochlear implants with co-injected human ESCs utilizing an animal model. Using an auditory neuropathy model, the animals demonstrated successful engrafting, differentiation, and improved auditory response thresholds using co-injected human ESCs with cochlear implants when compared with cochlear implants alone.
54
During cochlear implantation, exogenous factors are introduced to approximate or grow SGN processes, enhancing the contact between the neuron and the hair cell.
48,55,56
Although there is no definite evidence, it has been hypothesized that the success of a cochlear implant may depend on the health, number, and excitability of the SGNs.
49
When placing a cochlear implant, it is essential to minimize and eliminate iatrogenic damage to the SGNs. Multiple techniques have been studied to minimize iatrogenic damage including direct access to Rosenthal’s canal (where the cell bodies of SGNs are located), a translabyrinthine approach for direct access to the auditory nerve, and a cochleostomy into the scala tympani.
50
The cochleostomy of the scala tympani offered maximal survival of SGNs with minimal inflammation, while the invasive translabyrinthine approach induced the greatest inflammatory response and significantly reduced survival of the SGNs. It is extremely important to refine the techniques of cochlear implantation because as little as a 10% loss of neural tissue can lead to changes in the stimulation profile of the auditory nerve. This could in turn lead to inconsistencies in the expected results of the cochlear implants versus the achieved results.
44
In vivo and in vitro models of spiral ganglion neurons
Researchers have successfully injected MSCs into the scala tympani of mice subjected to acoustic trauma. It was observed that the stem cells migrated into Rosenthal’s canal and the organ of Corti. The researchers were able to accomplish this without the introduction of exogenous transcription factors or proteins. These results suggest that the extracellular environments of the perilymph and endolymph have governing factors that function in the differentiation and localization of stem cells.
36
Many in vitro models have been able to successfully regenerate SGNs from MSCs. Adult nasal MSCs, whose primary function is to renew the olfactory epithelium, have been shown to have broad anatomic potential beyond the superior regions of the nasal cavity where olfaction occurs.
57
A study showed that SGNs can be replaced with nasal tissue-derived MSCs after gentamicin-induced ototoxicity.
53
An exogenous stimulator of the Wnt/B-catenin pathway was used to promote robust neuronal differentiation of the MSCs. SGNs generated from nasal MSCs expressed neuronal proteins and were excitable in culture. It was demonstrated that these MSCs share similar gene expression profiles as the inner ear precursor cells including STRO-1 and Nestin.
57
Human ESCs have also been used to undergo directed differentiation toward SGN-like sensory neurons, with an 82% to 95% similar expression profile of native SGNs. These mimics were shown to extend neurites to the cochlear nucleus. To induce this differentiation and specificity to the SGN progenitor cells, researchers induced the expression of PAX genes.
58
They also checked and verified the generated spiral ganglion neuron’s ability to generate action potentials. This study was significant in its ability to differentiate the signaling pathways of early and late precursors in the human SGN lineage.
59
Bone MSCs have also been used to generate otic precursors with characteristic markers such as SOX2, PAX8, GATA3, and PAX2. The cells could then be further manipulated to follow a neuronal pathway or a sensory hair cell pathway.
60
Based on cell-based models, multiple factors have been shown to influence SGN survival and prevent age-related loss of SGNs. Exogenous brain-derived neurotrophic factor (BDNF) has been shown to help preserve SGNs better than ears treated with artificial perilymph.
46
A study successfully manipulated Schwann cells of auditory neurons to overexpress BDNF. This model significantly promoted SGN and auditory neuron survival over control samples.
61
Genetically modified Schwann cells may provide a key translational project in the clinical setting of SGN preservation.
49
Beyond BDNF, multiple factors have been shown to participate in the direct stimulation of the auditory nerve. A study elucidated that both insulin-like growth factor-1 (IGF-1) and insulin signaling pathways affected SGN survival at the systemic level. In contrast, it was observed that calcium signaling pathways and free radicals contributed to the loss of SGNs.42 Leukemia inhibitory factor appears to be important in generating the neural precursors of SGNs and in the regeneration of other neural stem cells.62 It has also been demonstrated that neurotrophins BDNF and NT3 are expressed in precursor otic cells and have been found to be important in the survival of stem cell-derived neurons including SGNs.62 The key models highlighting the generation of cell-based models of the SGN and innervation of the inner ear are highlighted in Table 3.
Table 3.
A summary of models of the innervation of the inner ear
Model
|
Findings
|
Ref.
|
In vitro SGN model
|
• SGNs do not rely solely on hair cells for stimulation for survival.
• SGN rely on neutrotrophin-3 and glial-derived neurotrophic factor from accessory and supporting cells.
|
63
|
• BDNF and NT3 are important in the survival of SGNs
• LIF is needed in the generation of SGN precursors.
|
62
|
• Nasal MSCs share similar gene expression profiles as the inner ear precursor cells, including STRO-1 and Nestin expression.
|
57
|
• Bone MSCs can be transformed into otic precursor cells and express neuronal or hair cell markers.
|
60
|
Ex vivo SGN model
|
• Extensive fenestrae within bony structures guide efferent nerve fibers through the modiolus.
• May be a potential site of damage during CI.
|
43
|
• SGN can be replaced with nasal tissue-derived MSCs after gentamicin-induced hair cell death using an activator of the Wnt /B-catenin pathway.
|
53
|
In silico SGN simulation study
|
• 10% loss of neural tissues within the auditory nerve leads to significant changes in signal conduction.
• May explain some of the variability in CI success.
|
44
|
In vivo SGN Model
|
• Insulin, steroid hormones, and IGF-1 pathways promote SGN survival while free radicals and the activation of calcium signaling pathways promote cell death.
|
42
|
• Without Schwann cells, SGNs form tvestibular ganglion neurons outside of their normal location in Rosenthal’s canal.
|
64
|
• Schwann cells send signals to SGNs to stop, proliferate, and grow within Rosenthal’s canal.
|
65
|
• EYA1 and SIX1 drive the neuronal developmental program in the mammalian inner ear
|
66
|
• Gli3 repressor is required for patterning of dorsal inner ear structures, whereas Gli2/3 activator is necessary for the ventral ear structures.
|
67
|
• Loss of Pax2 affects tissue patterning within the cochlear duct, generating inner ear models that lack spiral ganglion.
|
68
|
• SGNs can survive for months after the loss of inner hair cells.
• Hair cells are not necessary for SGN survival.
• Supporting cells of the organ of Corti are likely the source of SGN survival factors.
|
47
|
• IT injection of ESCs in animal model CI showed successful engrafting and better hearing response than control.
|
54
|
• BDNF preserved SGN survival better than artificial perilymph.
|
46
|
• Genetically engineered Schwann cells overexpressing BDNF model promoted SGN and auditory neuron survival.
|
61
|
• Long term administration of BDNF have a protective effect on SGNs.
|
49
|
In vivo and in vitro SGN model
|
• Stem cells injected into the scala tympani of mice migrate into the organ of Corti and Rosenthal’s canal without the introduction of exogenous transcription factors or proteins.
|
36
|
In vitro and ex vivo SGN model
|
• Induced expression of PAX genes caused human ESCs to undergo directed differentiation to spiral ganglion-like neurons.
|
59
|
SGN = spiral ganglion neuron; SHH = Sonic hedgehog signaling; ESC = embryonic stem cells; MSC = mesenchymal stem cells; BDNF = Brain derived neurotrophic factor; NT3 = neurotrophin-3; CI = cochlear implantation.
Generation of the extracellular matrix
The extracellular matrix (ECM) of the cochlea has many roles, including but not limited to structural support, regulation of extracellular ion homeostasis, and monitoring fluid filtration and inner ear pressure.
10
One of the principal structures of the ECM and an essential component to mimic in models is the basement membrane. The mammalian basement membrane is primarily made up of four ECM components: collagen IV, laminin, nidogen, and heparin sulfate proteoglycans.
69
Of these, collagen IV is the most common ECM protein, providing strength and rigidity to the basement membrane of the cochlea. The self-assembly of the collagen triple helix has been mimicked in biomaterials, with models becoming increasingly similar to the native structure.
70
Some in vitro studies have shown strong adhesion between synthetic collagen IV and laminin.
71
Alport syndrome, an X-linked mutation of collagen IV, is associated with malformation of collagen IV that commonly leads to a progressive, genetic hearing loss.
72
Laminin is the second most prominent protein of the ECM and provides the initial scaffolding for basement membrane construction. In gerbils, the intimate interaction between laminin and collagen IV was visualized in the basement membrane of the endothelium, epithelium, and SGNs of the inner ear.
73
The models generated with laminin and other native proteins cultivated in ultra-high viscous alginate have served as a suitable matrix for BDNF (Table 4).
74,75
Table 4.
A Summary of 3D models of the inner ear, inner ear organoids, and 3D cultures of the inner ear
Model
|
Structures replicated
|
Ref.
|
Inner ear organoid |
• Generated hair cells harboring inner ear sensory epithelium using hPSCs.
|
76
|
Inner ear organoid |
• Generated organoid of the inner ear using mouse ESCs with mechanosensitive hair cells.
• These hair cells mimicked two native subtypes of vestibular hair cells through a dynamic pattern of ion channel expression.
|
77
|
Inner ear 3D culture |
• Defined an artificial stem cell niche for the future formation of 3D inner ear structures.
|
78
|
Inner ear organoid and inner ear 3D culture |
• Differentiated a single stem cell aggregate into multiple otic vesicle-like structures.
• Vesicles developed into inner ear organoids with functional sensory epithelial cells and hair cells having electrical physiological properties.
|
4
|
• Generated inner ear tissue containing hair cells, supporting cells, and sensory-like neurons.
|
79
|
hPSCs = human pluripotent stem cells; FGF = fibroblast growth factor; BMP = bone morphogenetic protein; TGF = transforming growth factor; RNA = ribonucleic acid.
Nidogen, a sulfated glycoprotein, primarily functions as an ECM liner molecule. Studies have shown that while it is not essential in the overall architecture of the quiescent basement membrane, it is essential in tissue transition and cell-to-cell communication.
80,81
Models have revealed that it may also have a minor role in the axonal pathfinding and synaptic transmission processes.
80
Multiple ECM components fall within the category of heparin sulfate proteoglycans and their functions vary between structure and ionic homeostasis. Heparin sulfate proteoglycans primarily function as adherents in the ECM and have intimate roles in the receptor-signaling complex. Human neural stem cell models have revealed additional roles for heparin sulfate proteoglycans in proliferation, migration, differentiation, and cell survival in the inner ear and neural niche.
82
In addition to our growing understanding of the ECM, there have been great strides in the generation of biomatrix and iPSC models to produce distinguishable structures of the components of the ECM. A study reported the successful use of decellularized mice cochlea as a scaffold for inner ear tissue differentiation.
83
The model generated in this study possessed human Wharton jelly cells, components of the umbilical cord, implanted into the acellular cochlea. Researchers were able to generate many anatomically identifiable structures of the organ of Corti. They concluded that this is a preliminary step in understanding how the ECM supports the sensory epithelium. Using a similar model, another study reported the generation of the vascularized human cochlear scaffolds from postmortem donors that were tolerated in vivo and allowed cellular engraftment. These studies provide a basis for understanding the ECM’s role in the cochlea and translating it from the bench to the clinic.
Progressing from cell-based models of the inner ear to organoid-based models
To truly generate and represent diseases, the current efforts of the scientific community need to advance beyond a 2D stem cell-based model to a 3D model that closely mimics native physiology (Table 4). One of the most significant limitations of 2D cultures is the inability to accurately model disease, which in turn limits our ability to understand pathogensis.
78
3D organoid models incorporate multiple cell lines into one culture to mimic structures of the body. One of the newer revolutionary technologies is to create 3D structures that mimic organs, organoids, or organ-on-chip models, which can more closely mimic the physiology of the inner ear and related diseases.
78
However, there are challenges in generating organ-on-chip-models due to the pleiotropic effects of replicating the developmental stages of heterogeneous cell lines in coculture.
84
Instead of manipulating a single cell type at a time, advances in 3D models will allow us to truly recapitulate inner ear disorders. Although hurdles exist with these models, a study highlighted many ways that 3D cell culturing can move to the forefront of modeling inner ear pathology.
85
One of these 3D models is the organ-on-a-chip model. Organ-on-a-chip models are generated using microchip manufacturing methods that create continuously perfused chambers that enclose living cells to mimic tissue- and organ-level physiology.
86
There have been many key discoveries that can lead to the development of organ-on-a-chip models of the inner ear in future studies. In in vitro models, it is best when stem cells can be differentiated without biological and exogenous biochemical agents that can impose systemic effects. It has been shown that the use of groove pattern arrays and ridges can effectively and rapidly differentiate human ESCs into neurites without the use of any differentiation-inducing agents.
87
One study also highlighted the importance of recapitulating the sequential control of signaling pathways to transform aggregates of ESCs into non-neural, preplacode/otic placode regions.
3
In such a 3D model, it was possible to generate presumptive otic placodes of hair cells with functional stereocilia and a kinocilium that mimicked native mechanosensitive hair cells. In addition, the generation of the non-neural and preplacode ectoderm was an innovative approach to creating cell-based models for auditory disorders.
A study observed that ECM proteins added to culture dishes could promote the generation of inner ear cells and supporting cells as part of a pseudostratified epithelium. These cultures contain sensory-like neurons with derived hair cells termed inner ear organoids. This study was one of the first attempts to generate a scalable and reproducible in vitro model of inner ear sensory tissue.
79
Other studies have utilized cochlear explant cultures to reinnervate hair cells using human iPSCs, which showed superiority with a greater number of contact points when compared to using human ESCs (Figs. 4 and 5).
88
Figure 4.
Coculture of cochlear explants and hESC- and hiPSC-derived neural progenitors. (a) The light microscope image depicts the cochlear explant cocultured with a stem cell-derived neurosphere (NS). (b) The explant-only control obtained from P3 mice shows the normal innervation pattern of ANs after 1 DIV. (c–e) In cocultures, growth of hESC- and hiPSC-derived neural processes towards and along the rows of hair cells was observed. Scale bar = 50𝜇m, relevant for all the images. (f) The hiPSC-derived neural processes made contact with fewer hair cells compared to the hESCs. ∗𝑝 < 0.05. NS = not significant. Adapted with permission from Gunewardene et al.
88
Figure 4.
Coculture of cochlear explants and hESC- and hiPSC-derived neural progenitors. (a) The light microscope image depicts the cochlear explant cocultured with a stem cell-derived neurosphere (NS). (b) The explant-only control obtained from P3 mice shows the normal innervation pattern of ANs after 1 DIV. (c–e) In cocultures, growth of hESC- and hiPSC-derived neural processes towards and along the rows of hair cells was observed. Scale bar = 50𝜇m, relevant for all the images. (f) The hiPSC-derived neural processes made contact with fewer hair cells compared to the hESCs. ∗𝑝 < 0.05. NS = not significant. Adapted with permission from Gunewardene et al.
88
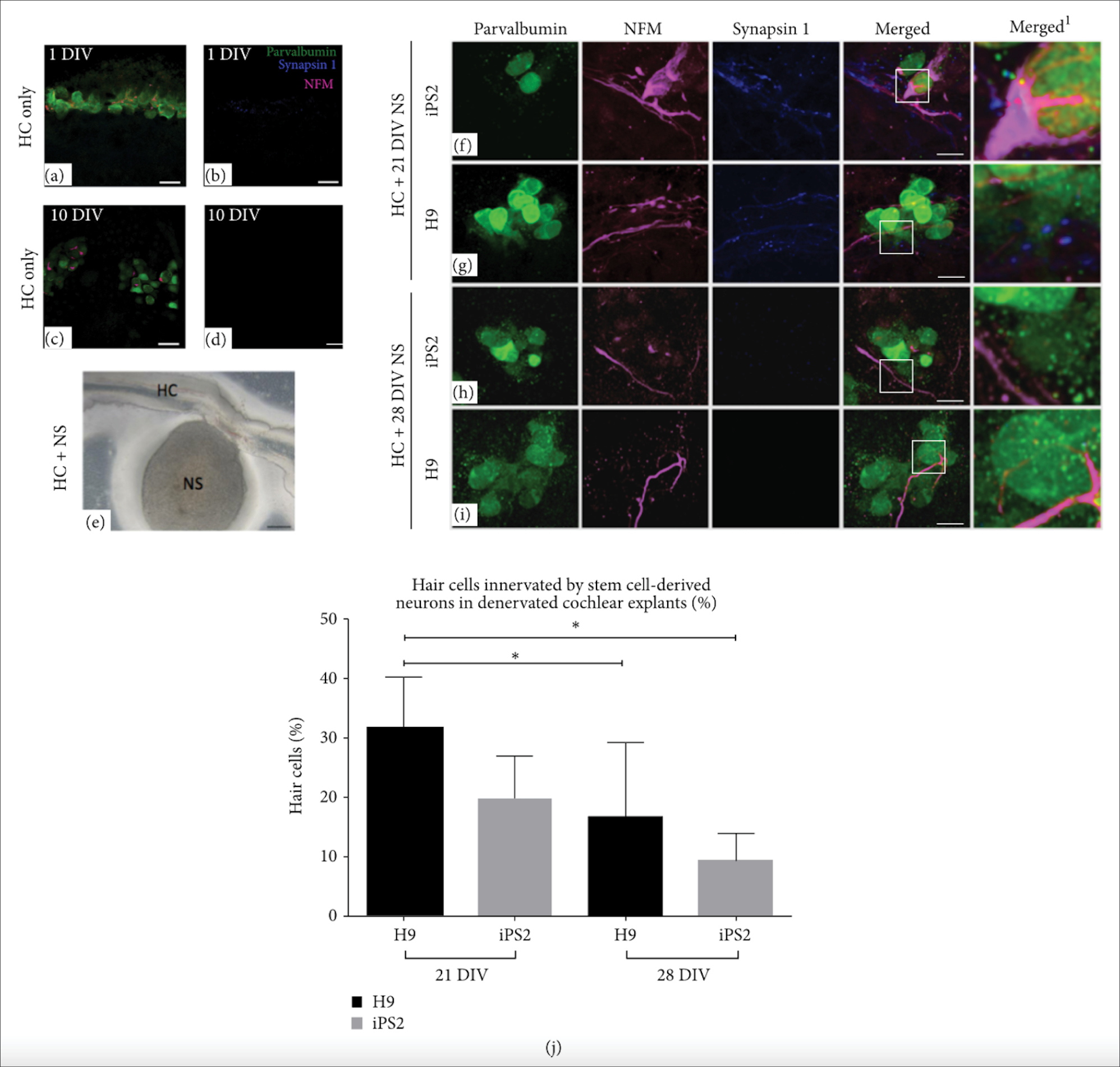
Figure 5.
The synaptic potential of 21- and 28-day-old hiPSC- and hESC-derived neurons. (a-b)
In the denervated cochlear explant controls at 1 DIV, some accumulation of residual NFM within hair cell somata and a few synaptic puncta were observed. (c-d) After 10 DIV, there appeared to be fewer hair cells with NFM accumulation and synapsin 1 was undetectable. (e) Light microscope image of denervated cochlear explant cocultured with stem cell-derived neurosphere. (f-g) The hiPSC and hESC neurospheres cocultured at 21 DIV extended their neural processes towards hair cells in the denervated explants. Punctate-like synapsin 1 expression was observed along the neural processes of both the hiPSC- and hESC-derived neurites making contact with hair cells. (h-i) The hiPSC and hESC neurospheres cocultured at 28 DIV projected fewer neural processes towards hair cells in the denervated explants. (j) The 21-day-old hiPSC- and hESC-derived neurons made contact with a significantly higher number of hair cells, compared to the 28-day-old neurons (H9: P < 0.05). *P < 0.05. Adapted with permission from Gunewardene et al.
88
Figure 5.
The synaptic potential of 21- and 28-day-old hiPSC- and hESC-derived neurons. (a-b)
In the denervated cochlear explant controls at 1 DIV, some accumulation of residual NFM within hair cell somata and a few synaptic puncta were observed. (c-d) After 10 DIV, there appeared to be fewer hair cells with NFM accumulation and synapsin 1 was undetectable. (e) Light microscope image of denervated cochlear explant cocultured with stem cell-derived neurosphere. (f-g) The hiPSC and hESC neurospheres cocultured at 21 DIV extended their neural processes towards hair cells in the denervated explants. Punctate-like synapsin 1 expression was observed along the neural processes of both the hiPSC- and hESC-derived neurites making contact with hair cells. (h-i) The hiPSC and hESC neurospheres cocultured at 28 DIV projected fewer neural processes towards hair cells in the denervated explants. (j) The 21-day-old hiPSC- and hESC-derived neurons made contact with a significantly higher number of hair cells, compared to the 28-day-old neurons (H9: P < 0.05). *P < 0.05. Adapted with permission from Gunewardene et al.
88
3D models of the inner ear have been shown to create the functional development of mechanosensitive hair cells. Mouse ESCs have been used to create an organoid of the inner ear that progressed through a sequential pattern of development of ion channel expression. This model closely mimicked the two native subtypes that are present in vestibular hair cells.
77
The researchers were able to generate organoids using self-guided development of ESCs into morphologically similar inner ear vestibular organs (Figs. 6 and 7).
Figure 6.
Inner ear organoids express multiple hair cell markers.
(a-b) The appearance of nGFP+ cells in the epithelium of organoid vesicles on day 12 of differentiation. (c) At later stages of differentiation (>day 14), numerous nGFP+ cells populated the epithelium, typically in a regional cluster as seen in d and e. (f-g) Most nGFP+ cells were also Anx4a+ and Calretinin (Calb2)+. Arrowheads in g denote nGFP+ cells devoid of Calb2. (h-i) nGFP+ hair cells had acetylated-tublin+ kinocilia and F-actin+ hair bundles. (j-k) Most Calb2+ hair cells also had Espin+ hair bundles. (l) nGFP+ cells were also Myo7a+ and Sox2+. Adapted with permission from Liu et al.
77
Figure 6.
Inner ear organoids express multiple hair cell markers.
(a-b) The appearance of nGFP+ cells in the epithelium of organoid vesicles on day 12 of differentiation. (c) At later stages of differentiation (>day 14), numerous nGFP+ cells populated the epithelium, typically in a regional cluster as seen in d and e. (f-g) Most nGFP+ cells were also Anx4a+ and Calretinin (Calb2)+. Arrowheads in g denote nGFP+ cells devoid of Calb2. (h-i) nGFP+ hair cells had acetylated-tublin+ kinocilia and F-actin+ hair bundles. (j-k) Most Calb2+ hair cells also had Espin+ hair bundles. (l) nGFP+ cells were also Myo7a+ and Sox2+. Adapted with permission from Liu et al.
77

Figure 7.
ES cell-derived organoids express biomarkers of Type I hair cells.
(a) The numerous Myo7a+, Calb2- hair cells were observed in a day 28 organoid (arrowheads). (b-c) High magnification image of a Myo7a+, Calb2- Type I-like hair cell (asterisk). (d–f) DIC images of organoid hair bundles with Type II- (d and e) and Type I- (f) like morphology. Type II- (e) and Type I- (f) like voltage-dependent currents were recorded from the cells at the center of these images (arrows). (g) Family of low-voltage-activated K+ currents, typical of vestibular Type I hair cells. (h) Instantaneous tail currents at −35 mV plotted as a function of prepulse voltage for three Type I-like organoid hair cells. (i) Membrane responses of an utricle Type I hair cell and a Type I-like organoid hair cell. (j) Family of membrane responses from a Type I-like organoid hair cell evoked by the current injection protocol shown (k) Summary timeline of the functional maturation of mechanotransduction and ion channel acquisition for utricle hair cells (blue) and organoid hair cells over time in culture (red). Adapted with permission from Liu et al.
77
Figure 7.
ES cell-derived organoids express biomarkers of Type I hair cells.
(a) The numerous Myo7a+, Calb2- hair cells were observed in a day 28 organoid (arrowheads). (b-c) High magnification image of a Myo7a+, Calb2- Type I-like hair cell (asterisk). (d–f) DIC images of organoid hair bundles with Type II- (d and e) and Type I- (f) like morphology. Type II- (e) and Type I- (f) like voltage-dependent currents were recorded from the cells at the center of these images (arrows). (g) Family of low-voltage-activated K+ currents, typical of vestibular Type I hair cells. (h) Instantaneous tail currents at −35 mV plotted as a function of prepulse voltage for three Type I-like organoid hair cells. (i) Membrane responses of an utricle Type I hair cell and a Type I-like organoid hair cell. (j) Family of membrane responses from a Type I-like organoid hair cell evoked by the current injection protocol shown (k) Summary timeline of the functional maturation of mechanotransduction and ion channel acquisition for utricle hair cells (blue) and organoid hair cells over time in culture (red). Adapted with permission from Liu et al.
77
To create proper organoid development, FGF, WNT, BMP, and TGF signaling were manipulated for the differentiation of a single stem cell aggregate into multiple otic vesicle-like structures.
4
Over the course of two months, the development of the vesicles into inner ear organoids was observed with functional sensory epithelial cells on the path to becoming sensory neurons. The induction of hair cells from the model also showed electrical physiological responses like native hair cells. This model was shown to produce an array of functional inner ear cell lines that could explain inner ear development and its repair mechanism. Recently, a protocol has been developed to differentiate human pluripotent stem cells (hPSCs) into a 3D, in vitro inner ear sensory epithelium with functional hair cells. This moves us one step closer to replicating the inner ear in vitro.
To advance that model, one research team has proposed the artificial stem cell niche that will be needed for the future 3D formation of inner ear structures.
78
It has been shown that the greater epithelial ridge cells are the principal organoid-forming progenitors of the mouse cochlea and can generate both the sensory hair cells and supporting cells.
89
Once normal physiologic organoids of the inner ear have been generated, there is a need to advance the models to mimic a diseased state. One study successfully generated inner ear organoids in a 3D culture by modulating the FGF, TGF, BMP, and WNT pathways in two human iPSCs.
4
In their model, it was possible to emulate the protein expression of a multitude of syndromic hearing loss disorders including Usher syndrome (USH1C, CDH23), Waardenburg Syndrome (SOX10), Pendred syndrome (SLC23A4), and Bartter syndrome (BSND). This opens the door to the evaluation of various inner ear pathologies using an organoid model. The delicate nature of the human inner ear makes biopsies near impossible to collect, and therefore the successful generation of human inner ear organoids could be an innovative and powerful platform to study human inner ear physiology, development, and disease.
76
Challenges in generating inner ear organoids
There are challenges with 3D modeling the inner ear. A common challenge appears to be mimicking the topography of the ECM and its functional components, which are crucial to mimic and drive desired stem cell differentiation.
The drainage pathways of the endolymph and perilymph within the ECM have been particularly difficult to model in vitro. Fortunately, different hydrogel-based scaffolds have been developed for the 3D cultivation of graft stem cells and the development of an organoid of the inner ear. These include hydrogel models such as bioprintable, cell-laden silk fibroid-gelatin hydrogels that are capable of culturing mesenchymal progenitor cells.
90,91
Many of these biomatrix models are self-assembling, and sonication has been shown to alter the hydrophobic interactions to help accelerate the assembly process from precursor molecules.
91
Beyond the structure of the ECM, there is also a need to mimic the topographic distribution. This includes the spatial differentiation of the ECM as well as the cellular components that can successfully generate 3D organoids. Technological advancements have led to the creation of biomaterials that nearly mimic the native ECM.
78
Increasing cell density within an ECM matrix has been shown to facilitate organoid formation. It was shown that cell-to-cell contact was more important to organoid development than diffusible signaling for inner ear cell types.
89
A final challenge of culturing organoids is that multiple cell lines, often with different culturing conditions, are being cultured at once. This makes it extremely difficult to include all required conditions in one model. However, a study introduced high-density semiconductor electrode arrays that enhanced survival, growth, neural orientation, morphology, and alignment of SGNs in vitro compared to a control. These developments, along with organ-on-a-chip and other models, have successfully cultured multiple cell lines in parallel.
79,92
It appears that many of the early challenges of generating inner ear organoids are being overcome, leading us closer to the mainstream use of these models in the elucidation of novel therapeutics and the pathophysiology of inner ear disease.
Conclusion and future directions
Cell-based models provide a powerful tool to understand the molecular mechanisms underlying the pathophysiology of auditory disorders as there are challenges to obtaining the cochlear tissue for scientific analyses. For the inner ear, this includes outer and inner hair cells, spiral ganglion neurons, and accessory cells including Hensen’s, Pillar, and Claudius cells. As we expand upon in vitro modeling techniques, we have been able to study both physiology and pathology of the inner ear. The most promising 2D models incorporate differentiated iPSCs, allowing the researcher to generate cell lines that are inherently difficult to harvest by using exogenous supplementation. The advantages of 2D in-vitro models are their high reproducibility, allowing for high-throughput drug-screening, and the highly controlled environment, allowing for the researcher to modify the temperature, oxygen, carbon dioxide, pH, and osmotic pressure. However, limitations to 2D models include the possibility of infection with microorganisms, cross-contamination with other cell lines, and the limited representation of normal physiology with the use of only a single cell line. 3D models help in circumventing some of these advantages of 2D models and more closely simulate the natural organization of tissues.
Organoids appear to be the best and most functional in vitro model for auditory disorders. Organoids offer another level of depth in approaching embryology and the etiology of both congenital and acquired diseases of the inner ear. They allow us to generate complex structures and tissues, such as the sensory hair cells with their underlying neuronal processes, that otherwise could not be regenerated. The inner ear organoids exhibit successful replication of the functionality of the inner ear including neuronal firing, stereocilia function, and synaptic transmission. Organoid development for the auditory system is relatively new but offers hope in the regeneration of multiple cell lines that are damaged throughout the course of these diseases. With each year we advance in our ability to generate cell-based models that more closely mimic in vivo physiology.
Through the expansion of organoid models, we are elucidating the function of the surrounding structures and the role they play in healthy, traumatized, and diseased states. For common diagnoses, such as SNHL, it is essential to go beyond replicating the hair cells and understand subsequent changes to the spiral ganglion neurons, supporting cells, and ECM that underlie the pathology. For the inner ear, we are seeing near complete replicas of the cochlea that can emulate the processing of therapeutics in vivo and their function. As cell-based models begin to evolve into more organoid and 3D-based approaches, we will eventually be able to closely mimic the pathophysiology of inner ear disease, further elucidate etiologies that underpin the phenotypic presentation of these diseases, and hopefully, advance novel drug therapies for various auditory diseases.
Acknowledgments
The research work in Dr. Eshraghi’s Laboratory is supported by translational grants from MED-EL Corporation and the HERA Foundation. We are grateful to Dr. Valerie Gramling for critical reading of the manuscript.
Funding sources
None.
Ethical statement
None to be declared.
Competing interests
HO is a senior member of the editorial board of the journal and YO is the editor-in-chief of the journal. The present article is an invited review that was carefully handled based on the publication/editorial policy and the established code of conduct of the journal.
Authors’ contribution
JK, AF, NBB, JM, HO, YO, RM and AAE performed the literature search, wrote the manuscript, critically reviewed, and revised the manuscript. All authors approved the final manuscript.
Review Highlights
What is the current knowledge?
√ Cell-based models of the inner ear have been able to mimic many of the physiologic processes of the inner ear.
√ Advancement from 2D to 3D models of the inner ear are novel in the field of otolaryngology.
What is new here?
√ The development of 3D models of the inner ear will allow researchers to further elucidate the pathophysiology of inner ear disorders.
√ Organoid models allow for novel drug elucidation and regrowth of cell lines lost in various inner ear disorders.
References
- Duval K, Grover H, Han LH, Mou Y, Pegoraro AF, Fredberg J. Modeling Physiological Events in 2D vs 3D Cell Culture. Physiology (Bethesda) 2017; 32:266-77. doi: 10.1152/physiol.00036.2016 [Crossref] [ Google Scholar]
- Vlasits AL, Simon JA, Raible DW, Rubel EW, Owens KN. Screen of FDA-approved drug library reveals compounds that protect hair cells from aminoglycosides and cisplatin. Hear Res 2012; 294:153-65. doi: 10.1016/j.heares.2012.08.002 [Crossref] [ Google Scholar]
- Koehler KR, Mikosz AM, Molosh AI, Patel D, Hashino E. Generation of inner ear sensory epithelia from pluripotent stem cells in 3D culture. Nature 2013; 500:217-21. doi: 10.1038/nature12298 [Crossref] [ Google Scholar]
- Koehler KR, Nie J, Longworth-Mills E, Liu XP, Lee J, Holt JR. Generation of inner ear organoids containing functional hair cells from human pluripotent stem cells. Nat Biotechnol 2017; 35:583-9. doi: 10.1038/nbt.3840 [Crossref] [ Google Scholar]
- Jeong M, O'Reilly M, Kirkwood NK, Al-Aama J, Lako M, Kros CJ. Generating inner ear organoids containing putative cochlear hair cells from human pluripotent stem cells. Cell Death Dis 2018; 9:922. doi: 10.1038/s41419-018-0967-1 [Crossref] [ Google Scholar]
- Duran Alonso MB, Feijoo-Redondo A, Conde de Felipe M, Carnicero E, García AS, García-Sancho J. Generation of inner ear sensory cells from bone marrow-derived human mesenchymal stem cells. Regen Med 2012; 7:769-83. doi: 10.2217/rme.12.65 [Crossref] [ Google Scholar]
- Mittal R, Woo FW, Castro CS, Cohen MA, Karanxha J, Mittal J. Organ-on-chip models: Implications in drug discovery and clinical applications. J Cell Physiol 2019; 234:8352-80. doi: 10.1002/jcp.27729 [Crossref] [ Google Scholar]
- Fritzsch B, Pan N, Jahan I, Elliott KL. Inner ear development: building a spiral ganglion and an organ of Corti out of unspecified ectoderm. Cell Tissue Res 2015; 361:7-24. doi: 10.1007/s00441-014-2031-5 [Crossref] [ Google Scholar]
- Freyer L, Aggarwal V, Morrow BE. Dual embryonic origin of the mammalian otic vesicle forming the inner ear. Development 2011; 138:5403-14. doi: 10.1242/dev.069849 [Crossref] [ Google Scholar]
- Whitfield TT. Development of the inner ear. Curr Opin Genet Dev 2015; 32:112-8. doi: 10.1016/j.gde.2015.02.006 [Crossref] [ Google Scholar]
- Moneta LB, Quintanilla-Dieck L. Embryology and anatomy of the ear. Operative Techniques in Otolaryngology-Head and Neck Surgery 2017; 28:66-71. doi: 10.1016/j.otot.2017.03.011 [Crossref] [ Google Scholar]
- Li–dong Z, Jun L, Yin–yan H, Jian–he S, Shi–ming Y. Supporting Cells–a New Area in Cochlear Physiology Study. Journal of Otology 2008; 3:9-17. doi: 10.1016/S1672-2930(08)50002-X [Crossref] [ Google Scholar]
- Hu Z, Ulfendahl M. The potential of stem cells for the restoration of auditory function in humans. Regen Med 2013; 8:309-18. doi: 10.2217/rme.13.32 [Crossref] [ Google Scholar]
- Chen W, Johnson SL, Marcotti W, Andrews PW, Moore HD, Rivolta MN. Human fetal auditory stem cells can be expanded in vitro and differentiate into functional auditory neurons and hair cell-like cells. Stem Cells 2009; 27:1196-204. doi: 10.1002/stem.62 [Crossref] [ Google Scholar]
- Chen W, Cacciabue-Rivolta DI, Moore HD, Rivolta MN. The human fetal cochlea can be a source for auditory progenitors/stem cells isolation. Hear Res 2007; 233:23-9. doi: 10.1016/j.heares.2007.06.006 [Crossref] [ Google Scholar]
- Menendez L, Trecek T, Gopalakrishnan S, Tao L, Markowitz AL, Haoze VY. Generation of inner ear hair cells by direct lineage conversion of primary somatic cells. elife 2020; 9:e55249. doi: 10.7554/eLife.55249 [Crossref] [ Google Scholar]
-
Tang P-C, Hashino E, Nelson RF. Progress in modeling and targeting inner ear disorders with pluripotent stem cells. Stem Cell Reports 2020. 10.1016/j.stemcr.2020.04.008
- Wiegand C, Banerjee I. Recent advances in the applications of iPSC technology. Curr Opin Biotechnol 2019; 60:250-8. doi: 10.1016/j.copbio.2019.05.011 [Crossref] [ Google Scholar]
- May LA, Kramarenko Kramarenko, II II, Brandon CS, Voelkel-Johnson C, Roy S, Truong K. Inner ear supporting cells protect hair cells by secreting HSP70. J Clin Invest 2013; 123:3577-87. doi: 10.1172/JCI68480 [Crossref] [ Google Scholar]
- Tritsch NX, Yi E, Gale JE, Glowatzki E, Bergles DE. The origin of spontaneous activity in the developing auditory system. Nature 2007; 450:50-5. doi: 10.1038/nature06233 [Crossref] [ Google Scholar]
- White PM, Doetzlhofer A, Lee YS, Groves AK, Segil N. Mammalian cochlear supporting cells can divide and trans-differentiate into hair cells. Nature 2006; 441:984-7. doi: 10.1038/nature04849 [Crossref] [ Google Scholar]
- Slepecky NB, Henderson CG, Saha S. Post-translational modifications of tubulin suggest that dynamic microtubules are present in sensory cells and stable microtubules are present in supporting cells of the mammalian cochlea. Hear Res 1995; 91:136-47. doi: 10.1016/0378-5955(95)00184-0 [Crossref] [ Google Scholar]
- Mammano F, Goodfellow S, Fountain E. Electrophysiological properties of Hensen's cells investigated in situ. Neuroreport 1996; 7:537-42. doi: 10.1097/00001756-199601310-00039 [Crossref] [ Google Scholar]
- Cohen R, Amir-Zilberstein L, Hersch M, Woland S, Loza O, Taiber S. Mechanical forces drive ordered patterning of hair cells in the mammalian inner ear. Nature communications 2020; 11:1-12. doi: 10.1038/s41467-020-18894-8 [Crossref] [ Google Scholar]
- Doetzlhofer A, Basch ML, Ohyama T, Gessler M, Groves AK, Segil N. Hey2 regulation by FGF provides a Notch-independent mechanism for maintaining pillar cell fate in the organ of Corti. Dev Cell 2009; 16:58-69. doi: 10.1016/j.devcel.2008.11.008 [Crossref] [ Google Scholar]
- Liu H, Chen L, Giffen KP, Stringham ST, Li Y, Judge PD. Cell-specific transcriptome analysis shows that adult pillar and Deiters' cells express genes encoding machinery for specializations of cochlear hair cells. Front Mol Neurosci 2018; 11:356. doi: 10.3389/fnmol.2018.00356 [Crossref] [ Google Scholar]
- Meenderink SW, Shera CA, Valero MD, Liberman MC, Abdala C. Morphological immaturity of the neonatal organ of Corti and associated structures in humans. Journal of the Association for Research in Otolaryngology 2019; 20:461-74. doi: 10.1007/s10162-019-00734-2 [Crossref] [ Google Scholar]
- Takumi Y, Nagelhus EA, Eidet J, Matsubara A, Usami Si, Shinkawa H. Select types of supporting cell in the inner ear express aquaporin-4 water channel protein. Eur J Neurosci 1998; 10:3584-95. doi: 10.1046/j.1460-9568.1998.00360.x [Crossref] [ Google Scholar]
- Cohen-Salmon M, Ott T, Michel V, Hardelin J-P, Perfettini I, Eybalin M. Targeted ablation of connexin26 in the inner ear epithelial gap junction network causes hearing impairment and cell death. Curr Biol 2002; 12:1106-11. doi: 10.1016/s0960-9822(02)00904-1 [Crossref] [ Google Scholar]
- Monzack EL, Cunningham LL. Lead roles for supporting actors: critical functions of inner ear supporting cells. Hear Res 2013; 303:20-9. doi: 10.1016/j.heares.2013.01.008 [Crossref] [ Google Scholar]
- Raphael Y, Altschuler RA. Structure and innervation of the cochlea. Brain Res Bull 2003; 60:397-422. doi: 10.1016/s0361-9230(03)00047-9 [Crossref] [ Google Scholar]
- Kelly MC, Chen P. Development of form and function in the mammalian cochlea. Curr Opin Neurobiol 2009; 19:395-401. doi: 10.1016/j.conb.2009.07.010 [Crossref] [ Google Scholar]
- Basch ML, Brown RM, 2nd 2nd, Jen HI, Groves AK. Where hearing starts: the development of the mammalian cochlea. J Anat 2016; 228:233-54. doi: 10.1111/joa.12314 [Crossref] [ Google Scholar]
- van der Valk WH, Steinhart MR, Zhang J, Koehler KR. Building inner ears: recent advances and future challenges for in vitro organoid systems. Cell Death Differ 2021; 28:24-34. doi: 10.1038/s41418-020-00678-8 [Crossref] [ Google Scholar]
- McClellan J, He W, Raja J, Stark G, Ren T, Reiss L. Effect of Cochlear Implantation on the Endocochlear Potential and Stria Vascularis. Otol Neurotol 2021; 42:e286-e93. doi: 10.1097/MAO.0000000000002949 [Crossref] [ Google Scholar]
- Parker MA, Corliss DA, Gray B, Anderson JK, Bobbin RP, Snyder EY. Neural stem cells injected into the sound-damaged cochlea migrate throughout the cochlea and express markers of hair cells, supporting cells, and spiral ganglion cells. Hear Res 2007; 232:29-43. doi: 10.1016/j.heares.2007.06.007 [Crossref] [ Google Scholar]
- Wangemann P, Nakaya K, Wu T, Maganti RJ, Itza EM, Sanneman JD. Loss of cochlear HCO3− secretion causes deafness via endolymphatic acidification and inhibition of Ca2+ reabsorption in a Pendred syndrome mouse model. American Journal of Physiology-Renal Physiology 2007; 292:F1345-F53. doi: 10.1152/ajprenal.00487.2006 [Crossref] [ Google Scholar]
- Zine A, Messat Y, Fritzsch B. A human induced pluripotent stem cell-based modular platform to challenge sensorineural hearing loss. Stem Cells 2021. doi: 10.1002/stem.3346 [Crossref]
- Nin F, Hibino H, Doi K, Suzuki T, Hisa Y, Kurachi Y. The endocochlear potential depends on two K+ diffusion potentials and an electrical barrier in the stria vascularis of the inner ear. Proceedings of the National Academy of Sciences 2008; 105:1751-6. doi: 10.1073/pnas.0711463105 [Crossref] [ Google Scholar]
- Locher H, de Groot JC, van Iperen L, Huisman MA, Frijns JH, Chuva de Sousa Lopes SM. Development of the stria vascularis and potassium regulation in the human fetal cochlea: Insights into hereditary sensorineural hearing loss. Dev Neurobiol 2015; 75:1219-40. doi: 10.1002/dneu.22279 [Crossref] [ Google Scholar]
- Giurdanella G, Montalbano G, Gennuso F, Brancati S, Lo Furno D, Augello A. Isolation, cultivation, and characterization of primary bovine cochlear pericytes: A new in vitro model of stria vascularis. J Cell Physiol 2019; 234:1978-86. doi: 10.1002/jcp.27545 [Crossref] [ Google Scholar]
- Bao J, Ohlemiller KK. Age-related loss of spiral ganglion neurons. Hear Res 2010; 264:93-7. doi: 10.1016/j.heares.2009.10.009 [Crossref] [ Google Scholar]
- Glueckert R, Pfaller K, Kinnefors A, Rask-Andersen H, Schrott-Fischer A. The human spiral ganglion: new insights into ultrastructure, survival rate and implications for cochlear implants. Audiol Neurootol 2005; 10:258-73. doi: 10.1159/000086000 [Crossref] [ Google Scholar]
- Sriperumbudur KK, Appali R, Gummer AW, van Rienen U. Neural Tissue Degeneration in Rosenthal's Canal and Its Impact on Electrical Stimulation of the Auditory Nerve by Cochlear Implants: An Image-Based Modeling Study. Int J Mol Sci 2020; 21. doi: 10.3390/ijms21228511 [Crossref]
- Fuchs JC, Tucker AS. Development and Integration of the Ear. Curr Top Dev Biol 2015; 115:213-32. doi: 10.1016/bs.ctdb.2015.07.007 [Crossref] [ Google Scholar]
- Miller JM, Chi DH, O'Keeffe LJ, Kruszka P, Raphael Y, Altschuler RA. Neurotrophins can enhance spiral ganglion cell survival after inner hair cell loss. Int J Dev Neurosci 1997; 15:631-43. doi: 10.1016/s0736-5748(96)00117-7 [Crossref] [ Google Scholar]
- Zilberstein Y, Liberman MC, Corfas G. Inner hair cells are not required for survival of spiral ganglion neurons in the adult cochlea. J Neurosci 2012; 32:405-10. doi: 10.1523/JNEUROSCI.4678-11.2012 [Crossref] [ Google Scholar]
- Roehm PC, Hansen MR. Strategies to preserve or regenerate spiral ganglion neurons. Curr Opin Otolaryngol Head Neck Surg 2005; 13:294-300. doi: 10.1097/01.moo.0000180919.68812.b9 [Crossref] [ Google Scholar]
- Scheper V, Hoffmann A, Gepp MM, Schulz A, Hamm A, Pannier C. Stem Cell Based Drug Delivery for Protection of Auditory Neurons in a Guinea Pig Model of Cochlear Implantation. Front Cell Neurosci 2019; 13:177. doi: 10.3389/fncel.2019.00177 [Crossref] [ Google Scholar]
- Backhouse S, Coleman B, Shepherd R. Surgical access to the mammalian cochlea for cell-based therapies. Exp Neurol 2008; 214:193-200. doi: 10.1016/j.expneurol.2008.08.002 [Crossref] [ Google Scholar]
- Eshraghi AA, Nazarian R, Telischi FF, Rajguru SM, Truy E, Gupta C. The cochlear implant: historical aspects and future prospects. The Anatomical Record Advances in Integrative Anatomy and Evolutionary Biology 2012; 295:1967-80. doi: 10.1002/ar.22580 [Crossref] [ Google Scholar]
- Carlson ML. Cochlear implantation in adults. N Engl J Med 2020; 382:1531-42. doi: 10.1056/NEJMra1904407 [Crossref] [ Google Scholar]
- Bas E, Van De Water TR, Lumbreras V, Rajguru S, Goss G, Hare JM. Adult human nasal mesenchymal-like stem cells restore cochlear spiral ganglion neurons after experimental lesion. Stem Cells Dev 2014; 23:502-14. doi: 10.1089/scd.2013.0274 [Crossref] [ Google Scholar]
- Chen W, Jongkamonwiwat N, Abbas L, Eshtan SJ, Johnson SL, Kuhn S. Restoration of auditory evoked responses by human ES-cell-derived otic progenitors. Nature 2012; 490:278-82. doi: 10.1038/nature11415 [Crossref] [ Google Scholar]
- Li CY, Mittal R, Bergman J, Mittal J, Eshraghi AA. Recent advancements toward gapless neural-electrode interface post-cochlear implantation. Neural Regeneration Research 2021; 16:1805. doi: 10.4103/1673-5374.306085 [Crossref] [ Google Scholar]
- Eshraghi AA, Nguyen D, Mittal R. Can Brain-Derived Neurotrophic Factor Therapy Improve Clinical Outcomes of Cochlear Implantation?. JAMA Otolaryngology–Head & Neck Surgery 2018; 144:287-8. doi: 10.1001/jamaoto.2017.3414 [Crossref] [ Google Scholar]
- Goldstein BJ, Hare JM, Lieberman S, Casiano R. Adult human nasal mesenchymal stem cells have an unexpected broad anatomic distribution. Int Forum Allergy Rhinol 2013; 3:550-5. doi: 10.1002/alr.21153 [Crossref] [ Google Scholar]
- Grocott T, Tambalo M, Streit A. The peripheral sensory nervous system in the vertebrate head: a gene regulatory perspective. Dev Biol 2012; 370:3-23. doi: 10.1016/j.ydbio.2012.06.028 [Crossref] [ Google Scholar]
- Matsuoka AJ, Morrissey ZD, Zhang C, Homma K, Belmadani A, Miller CA. Directed Differentiation of Human Embryonic Stem Cells Toward Placode-Derived Spiral Ganglion-Like Sensory Neurons. Stem Cells Transl Med 2017; 6:923-36. doi: 10.1002/sctm.16-0032 [Crossref] [ Google Scholar]
- Boddy SL, Chen W, Romero-Guevara R, Kottam L, Bellantuono I, Rivolta MN. Inner ear progenitor cells can be generated in vitro from human bone marrow mesenchymal stem cells. Regen Med 2012; 7:757-67. doi: 10.2217/rme.12.58 [Crossref] [ Google Scholar]
- Pettingill LN, Wise AK, Geaney MS, Shepherd RK. Enhanced auditory neuron survival following cell-based BDNF treatment in the deaf guinea pig. PLoS One 2011; 6:e18733. doi: 10.1371/journal.pone.0018733 [Crossref] [ Google Scholar]
- Oshima K, Teo DT, Senn P, Starlinger V, Heller S. LIF promotes neurogenesis and maintains neural precursors in cell populations derived from spiral ganglion stem cells. BMC Dev Biol 2007; 7:112. doi: 10.1186/1471-213X-7-112 [Crossref] [ Google Scholar]
- Zheng JL, Stewart RR, Gao W. Neurotrophin-4/5 enhances survival of cultured spiral ganglion neurons and protects them from cisplatin neurotoxicity. J Neurosci 1995; 15:5079-87. doi: 10.1523/JNEUROSCI.15-07-05079.1995 [Crossref] [ Google Scholar]
- Mao Y, Reiprich S, Wegner M, Fritzsch B. Targeted deletion of Sox10 by Wnt1-cre defects neuronal migration and projection in the mouse inner ear. PLoS One 2014; 9:e94580. doi: 10.1371/journal.pone.0094580 [Crossref] [ Google Scholar]
- Yang T, Kersigo J, Jahan I, Pan N, Fritzsch B. The molecular basis of making spiral ganglion neurons and connecting them to hair cells of the organ of Corti. Hear Res 2011; 278:21-33. doi: 10.1016/j.heares.2011.03.002 [Crossref] [ Google Scholar]
- Ahmed M, Xu J, Xu P-X. EYA1 and SIX1 drive the neuronal developmental program in cooperation with the SWI/SNF chromatin-remodeling complex and SOX2 in the mammalian inner ear. Development 2012; 139:1965-77. doi: 10.1242/dev.071670 [Crossref] [ Google Scholar]
- Bok J, Dolson DK, Hill P, Rüther U, Epstein DJ, Wu DK. Opposing gradients of Gli repressor and activators mediate Shh signaling along the dorsoventral axis of the inner ear. Development 2007; 134:1713-22. doi: 10.1242/dev.000760 [Crossref] [ Google Scholar]
- Burton Q, Cole LK, Mulheisen M, Chang W, Wu DK. The role of Pax2 in mouse inner ear development. Dev Biol 2004; 272:161-75. doi: 10.1016/j.ydbio.2004.04.024 [Crossref] [ Google Scholar]
- Ishiyama A, Mowry SE, Lopez IA, Ishiyama G. Immunohistochemical distribution of basement membrane proteins in the human inner ear from older subjects. Hear Res 2009; 254:1-14. doi: 10.1016/j.heares.2009.03.014 [Crossref] [ Google Scholar]
- Sorushanova A, Delgado LM, Wu Z, Shologu N, Kshirsagar A, Raghunath R. The collagen suprafamily: from biosynthesis to advanced biomaterial development. Advanced materials 2019; 31:1801651. doi: 10.1002/adma.201801651 [Crossref] [ Google Scholar]
- Nugroho RWN, Harjumäki R, Zhang X, Lou Y-R, Yliperttula M, Valle-Delgado JJ. Quantifying the interactions between biomimetic biomaterials–collagen I, collagen IV, laminin 521 and cellulose nanofibrils–by colloidal probe microscopy Colloids and Surfaces B. Biointerfaces 2019; 173:571-80. doi: 10.1016/j.colsurfb.2018.09.073 [Crossref] [ Google Scholar]
- Nozu K, Nakanishi K, Abe Y, Udagawa T, Okada S, Okamoto T. A review of clinical characteristics and genetic backgrounds in Alport syndrome. Clin Exp Nephrol 2019; 23:158-68. doi: 10.1007/s10157-018-1629-4 [Crossref] [ Google Scholar]
- Sakaguchi N, Spicer SS, Thomopoulos GN, Schulte BA. Increased laminin deposition in capillaries of the stria vascularis of quiet-aged gerbils. Hear Res 1997; 105:44-56. doi: 10.1016/s0378-5955(96)00180-3 [Crossref] [ Google Scholar]
- Swindle-Reilly KE, Papke JB, Kutosky HP, Throm A, Hammer JA, Harkins AB. The impact of laminin on 3D neurite extension in collagen gels. Journal of neural engineering 2012; 9:046007. doi: 10.1088/1741-2560/9/4/046007 [Crossref] [ Google Scholar]
- Hütten M, Erhacrt F, Zimmermann H, Reich U, Esser K-H, Lenarz T. UHV-alginate as matrix for neurotrophic factor producing cells—a novel biomaterial for cochlear implant optimization to preserve inner ear neurons from degeneration. Otol Neurotol 2013; 34:1127-33. doi: 10.1097/MAO.0b013e3182804949 [Crossref] [ Google Scholar]
- Nie J, Hashino E. Generation of inner ear organoids from human pluripotent stem cells. Methods Cell Biol 2020; 159:303-21. doi: 10.1016/bs.mcb.2020.02.006 [Crossref] [ Google Scholar]
- Liu XP, Koehler KR, Mikosz AM, Hashino E, Holt JR. Functional development of mechanosensitive hair cells in stem cell-derived organoids parallels native vestibular hair cells. Nat Commun 2016; 7:11508. doi: 10.1038/ncomms11508 [Crossref] [ Google Scholar]
- de Groot SC, Sliedregt K, van Benthem PPG, Rivolta MN, Huisman MA. Building an Artificial Stem Cell Niche: Prerequisites for Future 3D-Formation of Inner Ear Structures-Toward 3D Inner Ear Biotechnology. Anat Rec (Hoboken) 2020; 303:408-26. doi: 10.1002/ar.24067 [Crossref] [ Google Scholar]
- Koehler KR, Hashino E. 3D mouse embryonic stem cell culture for generating inner ear organoids. Nat Protoc 2014; 9:1229-44. doi: 10.1038/nprot.2014.100 [Crossref] [ Google Scholar]
- Ho MS, Böse K, Mokkapati S, Nischt R, Smyth N. Nidogens—Extracellular matrix linker molecules. Microsc Res Tech 2008; 71:387-95. doi: 10.1002/jemt.20567 [Crossref] [ Google Scholar]
- Vasudevan A, Ho MS, Weiergräber M, Nischt R, Schneider T, Lie A. Basement membrane protein nidogen-1 shapes hippocampal synaptic plasticity and excitability. Hippocampus 2010; 20:608-20. doi: 10.1002/hipo.20660 [Crossref] [ Google Scholar]
- Peall IW, Okolicsanyi RK, Griffiths LR, Haupt LM. Three-Dimensional Human Neural Stem Cell Models to Mimic Heparan Sulfate Proteoglycans and the Neural Niche. Semin Thromb Hemost 2021; 47:308-15. doi: 10.1055/s-0041-1724117 [Crossref] [ Google Scholar]
- Mellott AJ, Shinogle HE, Nelson-Brantley JG, Detamore MS, Staecker H. Exploiting decellularized cochleae as scaffolds for inner ear tissue engineering. Stem Cell Res Ther 2017; 8:1-9. doi: 10.1186/s13287-017-0505-6 [Crossref] [ Google Scholar]
- Fritzsch B, Beisel K. Development and maintenance of ear innervation and function: lessons from mutations in mouse and man. Am J Hum Genet 1998; 63:1263-70. doi: 10.1086/302126 [Crossref] [ Google Scholar]
- Akkerman N, Defize LH. Dawn of the organoid era: 3D tissue and organ cultures revolutionize the study of development, disease, and regeneration. Bioessays 2017; 39. doi: 10.1002/bies.201600244 [Crossref]
- Bhatia SN, Ingber DE. Microfluidic organs-on-chips. Nat Biotechnol 2014; 32:760-72. doi: 10.1038/nbt.2989 [Crossref] [ Google Scholar]
- Lee MR, Kwon KW, Jung H, Kim HN, Suh KY, Kim K. Direct differentiation of human embryonic stem cells into selective neurons on nanoscale ridge/groove pattern arrays. Biomaterials 2010; 31:4360-6. doi: 10.1016/j.biomaterials.2010.02.012 [Crossref] [ Google Scholar]
- Gunewardene N, Crombie D, Dottori M, Nayagam BA. Innervation of cochlear hair cells by human induced pluripotent stem cell-derived neurons in vitro. Stem cells international 2016; 2016. doi: 10.1155/2016/1781202 [Crossref]
- Kubota M, Scheibinger M, Jan TA, Heller S. Greater epithelial ridge cells are the principal organoid-forming progenitors of the mouse cochlea. Cell Rep 2021; 34:108646. doi: 10.1016/j.celrep.2020.108646 [Crossref] [ Google Scholar]
- Banerjee A, Arha M, Choudhary S, Ashton RS, Bhatia SR, Schaffer DV. The influence of hydrogel modulus on the proliferation and differentiation of encapsulated neural stem cells. Biomaterials 2009; 30:4695-9. doi: 10.1016/j.biomaterials.2009.05.050 [Crossref] [ Google Scholar]
- Das S, Pati F, Choi YJ, Rijal G, Shim JH, Kim SW. Bioprintable, cell-laden silk fibroin-gelatin hydrogel supporting multilineage differentiation of stem cells for fabrication of three-dimensional tissue constructs. Acta Biomater 2015; 11:233-46. doi: 10.1016/j.actbio.2014.09.023 [Crossref] [ Google Scholar]
- Radotic V, Braeken D, Drvis P, Mattotti M, Kovacic D. Advantageous environment of micro-patterned, high-density complementary metal-oxide-semiconductor electrode array for spiral ganglion neurons cultured in vitro. Sci Rep 2018; 8:7446. doi: 10.1038/s41598-018-25814-w [Crossref] [ Google Scholar]