Bioimpacts. 15:30454.
doi: 10.34172/bi.30454
Original Article
Platelet-derived microparticles enhance Ara-C-induced cell death in acute lymphoblastic leukemia (Nalm-6)
Fariba Nikravesh Formal analysis, Investigation, Methodology, Project administration, Writing – original draft, Writing – review & editing, 1, # 
Hossein Arezoomand Investigation, Methodology, Writing – original draft, Writing – review & editing, 1, # 
Roohollah h Mirzaee Khalilabadi Writing – review & editing, 1
Maryam Nooshadokht Investigation, 2
Hajar Mardani Valandani Conceptualization, Funding acquisition, Methodology, Project administration, Supervision, Writing – review & editing, 1, * 
Author information:
1Department of Hematology and Laboratory Sciences, Faculty of Allied Medicine, Kerman University of Medical Sciences, Kerman, Iran
2Department of Medical Parasitology and Mycology, School of Medicine, Kerman University of Medical Sciences, Kerman, Iran
#These authors contributed equally in this project.
Abstract
Introduction:
The current understanding highlights the intricate relationship between leukemic cells and their microenvironment, emphasizing the significant impact of environmental factors on chemotherapy resistance or sensitivity. Platelet-derived microparticles (PMPs) play a crucial role in facilitating intercellular communication, significantly contributing to the complex dynamics of cancer pathology and treatment outcomes. This study aims to investigate the cytotoxic and apoptotic effects of PMP, Ara-C, and their combinations on cancer cells, as well as their influence on the expression of critical genes like Bax, Bcl-2, P21, and h-TERT in the context of Acute Lymphoblastic Leukemia (ALL) cell line (Nalm-6).
Methods:
PMPs were isolated through centrifugation at varying speeds, and their concentration was determined using the BCA assay. The size and immunophenotypic characteristics of PMPs were analyzed using dynamic light scattering (DLS) and flow cytometry. The cytotoxic and apoptotic effects of PMP, Ara-C, and their combinations on Nalm-6 cells were assessed using the MTT assay, the trypan blue exclusion assay, and flow cytometry. Gene expression levels were analyzed using real-time PCR.
Results:
According to our research findings, PMPs did not independently impact the viability and apoptosis of Nalm-6 cells; however, they synergistically augmented Ara-C's suppressive impact on viability and apoptosis. The MTT assay showed that both PMPs and Ara-C, whether administered alone or in combination, had a cytotoxic effect on the Nalm-6 cells. Furthermore, the combined treatment significantly affected the expression of Bax, Bcl-2, P21, and h-TERT genes.
Conclusion:
Our study demonstrates that PMPs have the potential to improve the effectiveness of Ara-C chemotherapy in treating ALL. These findings contribute to a deeper understanding of the interplay between PMP and chemotherapy agents, offering potential insights for optimizing treatment strategies and improving patient outcomes in ALL.
Keywords: Acute Lymphoblastic Leukemia, Platelet-derived Microparticle, Cytarabine, Leukemia microenvironment, Platelet
Copyright and License Information
© 2025 The Author(s).
This work is published by BioImpacts as an open access article distributed under the terms of the Creative Commons Attribution Non-Commercial License (
http://creativecommons.org/licenses/by-nc/4.0/). Non-commercial uses of the work are permitted, provided the original work is properly cited.
Funding Statement
This study was supported by the Vice Chancellor for Research and Technology of Kerman University of Medical Sciences (Grant number 400001101). The authors have no relevant financial or non-financial interests to disclose.
Introduction
Acute lymphoblastic leukemia (ALL) is a fast-growing blood cancer caused by chromosome abnormalities and genetic changes that impact the growth and quick division of immature T or B cells.1 These genetic changes lead to the accumulation of leukemic cells in the bone marrow and other tissues, suppressing normal hematopoiesis in the bone marrow.2,3 ALL accounts for 75% of leukemia cases in children and is the third most common type of leukemia in adults. While ALL has a favorable prognosis and survival rates (around 80%) in children, it represents a severe condition in adults, with a survival rate below 40%.4 Chemotherapy in ALL consists of three phases: induction, consolidation, and maintenance.5 Ara-C is an effective chemotherapy drug for the treatment of ALL. This drug is often used in combination chemotherapy along with fluorouracil, methotrexate, and dexamethasone in solid tumors and leukemia.6 The impact of Ara-C stems from its active absorption into specific cells and subsequent conversion to its active form through metabolism. The rapid inactivation of Ara-C by plasma enzymes makes its plasma half-life about 10 minutes. As a result, for more effectiveness, Ara-C is prescribed in high doses.7 While low-dose Ara-C therapy is generally well tolerated, significant toxicities mainly occur with high-dose treatment. The dose-dependent toxicity is a notable limitation of Ara-C therapy.8 The objectives of ongoing ALL studies aim to optimize treatment strategies with less toxic interventions, enhance survival outcomes, and improve the overall quality of life for patients.9 Previous studies have demonstrated that, besides the dosage and combination of chemotherapy drugs, environmental factors significantly impact cancer cell biology and influence the overall effectiveness of cancer treatment.1 Today, it is known that malignancies, including leukemia, are closely related to their environment, and platelets are an essential part of the cancer cell microenvironment.10 Studies demonstrate that cancer cells activate platelets, leading to the release of platelet granule contents and microparticles known as platelet-derived microparticles (PMPs).11 PMPs are plasma membrane particles released from activated platelets during membrane budding. These particles constitute over 90% of microparticles found in the bloodstream.12 PMPs, which carry various functional compounds of platelets, serve as potential mediators between platelets and cancer cells. PMPs are an especially interesting type of extracellular vesicles for evaluating cancer, as their increase has been observed in all cancer types, including stomach, lung, skin, CML, CLL, ALL, and others.13 PMPs affect growth and proliferation, apoptosis, and gene expression patterns in the target cells by transferring genetic and biochemical content.14-16 These findings show that PMPs can affect the biology of acute lymphoid leukemia cells and the effectiveness of chemotherapy drugs, improving risk-based treatments and reducing the dose and toxicity of drugs. Although various studies have investigated the dual effect of PMP in the progression and biology of cancer cells, the current knowledge in this field seems incomplete. The Previous study shows that Ara-C's impact on the expression of Bax, Bcl-2, P21, and h-TERT genes is crucial in its anti-cancer mechanism. It involves regulating the cell cycle, inducing apoptosis, and maintaining telomeres. Ara-C can boost the expression of proapoptotic genes like Bax, caspase-9, and caspase-3 at both mRNA and protein levels while suppressing the expression of the anti-apoptotic gene Bcl-2 in renal carcinoma cells. Experiments have proven that Ara-C can impede the AKT/PI3K signaling pathway's activity, thereby promoting cell apoptosis.17-20 In summary, while previous studies have investigated the role of PMPs in cancer biology and chemotherapy response, our study aims to provide novel insights into the specific mechanisms underlying the interaction between PMPs and cancer cells, particularly in the context of Ara-C treatment for ALL. By examining the impact of PMPs, Ara-C, and their combination on critical parameters such as cell survival, apoptosis induction, and gene expression profiles such as Bax, Bcl-2, P21, and h-TERT. We hypothesize that PMPs enhance the apoptotic effects of Ara-C and modulate the expression of critical genes involved in cell survival and proliferation pathways. These findings may contribute to developing more effective and targeted therapies for ALL.
Materials and Methods
Preparation of platelet concentrates
Kerman Blood Transfusion Center provided expired platelet concentrate (PC) bags.
Separation of PMPs from PCs
A multi-step centrifugation technique was used to separate PMPs from PCs. To separate the cells (RBCs and WBCs), the PCs were transferred into 50 mL Falcon tubes and centrifuged at 2500 g for 10 minutes. This process was repeated to ensure the complete removal of any remaining cells. The resulting supernatant solution underwent centrifugation at 22000 g for 30 minutes to precipitate the PMP. The isolated PMP was then washed with PBS to eliminate any residual plasma and stored at -70 °C for future use.21
Characterization of isolated PMPs
Determination of the size of PMPs
Dynamic light scattering (DLS) is a scientific technique used to measure the dimensions and size distribution of particles or molecules in a liquid or solution. DLS relies on the concept that particles in a liquid undergo random, thermally induced movements known as Brownian motion. These movements cause fluctuations in the intensity of scattered laser light. During a DLS experiment, a sample containing particles is exposed to a focused laser beam, and a detector records the changes in scattered light intensity over time at a specific angle. The software integrated into the device is employed to determine the average size of the particles.
The Malvern Master Sizer 2000 laser diffraction apparatus, utilizing DLS technology, is utilized for sizing analysis. Within this setup, a laser beam with a 633 nm wavelength is aimed at the suspension housing microparticles. Subsequently, the scattered light is captured, and the instrument's software computes the dimensions of the microparticles.22
Examining the surface marker of PMPs
Flow cytometry was used to validate the separation technique and confirm the cellular origin of the isolated PMPs by identifying platelet-specific markers CD42b and CD61 on the surface of the PMPs. 250 μL of PMP suspension was mixed with an equal volume of PBS (1:1 ratio in PBS). Subsequently, 100 µL of the diluted PMP solution was combined with two test tubes and two control tubes, each containing 10 µL of CD42b and CD61 antibodies, as well as 10 µL of IgG-FITC/PE antibody targeting mouse cells. After a thirty-minute incubation period at room temperature in the dark, the samples were analyzed using a flow cytometry instrument from BD Bioscience, with data management carried out using FLOMAX software.23
Determining the concentration of PMPs
The BCA method, which has been endorsed by the International Society of Extracellular Vesicles (ISEV), is a reliable technique for quantifying protein concentrations in various samples, including PMPs.24 The approach employed in this technique is based on the ability of proteins to convert copper ions into a colored chelate complex. This complex can then be measured using spectrophotometry to determine its quantity. The total protein content of PMPs was assessed using the Micro BCA Protein Assay Kit (Pierce, Thermo Scientific, Rockford, IL, USA) by the manufacturer's guidelines. Bovine serum albumin (BSA) served as the standard for comparative purposes.21
Cell culture
The Nalm-6 cell line (Nalm-6, NCBI C212) was acquired from the Pasteur Institute collection located in Tehran, Iran. The Nalm-6 cells were cultured in RPMI-1640 medium, which was supplemented with 10% fetal bovine serum (FBS) (GibcoTM A3160402) and 1% antibiotic (Penicillin-Streptomycin Solution 100X, Biowest, L0022). Subsequently, the cells were incubated in a humidified incubator at a temperature of 37 °C with a 5% CO2 atmosphere.25
Calculation of the combination index and the dose reduction index
CI (combination index) between PMP and Ara-C was determined using CompuSyn Software (ComboSyn, Inc., Paramus, NJ, USA). CI to quantitatively depict synergism (CI < 1), additive effect (CI = 1), and antagonism (CI > 1).
The dose reduction index (DRI) quantifies how much the dosage of a combination can be decreased to attain a particular level of effect compared to the concentration of the individual drug used alone.26
Trypan Blue assay
Nalm-6 cells were cultured in a 6-well plate at a density of 5 × 105 cells per well. The cells were then treated with different concentrations of PMP (200, 400, and 800 µg/mL) and Ara-C (0.5, 1, 1.5, and 2 µM) for 24, 48, and 72 hours to evaluate the apoptotic effect of these compounds on cell viability. The cell suspension underwent centrifugation, followed by placing the pellet in a serum-free complete medium. Subsequently, a mixture consisting of one part 0.4% trypan blue (GibcoTM 15250061) and one part cell suspension was incubated for 2 minutes at room temperature. The total number of unstained (viable) and stained (non-viable) cells was manually counted using a Neuberger chamber and a light microscope (ECLIPSE E100, Nikon). Finally, the percentage of viable cells was determined by calculating "Viability (%) = viable cells / viable cells + death cells × 100".27
MTT assay
The MTT colorimetric assay was utilized to assess the cytotoxicity of PMP, Ara-C, and their combinations against cancer cell lines in vitro. This assay measures the capacity of viable cells to convert yellow tetrazolium salts into blue-colored formazan crystals. Nalm-6 cells were seeded at a density of 1 × 104 cells per well in 96-well plates and exposed to varying concentrations of PMP (200, 400, and 800 µg/mL) and Ara-C (1 and 1.5 µM) for durations of 24, 48, and 72 hours. Furthermore, viability evaluations were carried out for combinations of PMP/Ara-C, such as PMP (200 µg/mL) + Ara-C (1 µM), PMP (200 µg/mL) + Ara-C (1.5 µM), PMP (400 µg/mL) + Ara-C (1 µM), and PMP (400 µg/mL) + Ara-C (1.5 µM). The plate was then centrifuged at 700g for 10 minutes, and the supernatant was removed. The cells were then exposed to 100 µL MTT solution (0.5 mg/mL; (M5655, Sigma) at 37 °C. After 4 hours, the colored formazan was dissolved in each well with 150 µL DMSO, and the optical absorbance at 570 nm was gauged using an enzyme-linked immunosorbent assay (ELISA). The metabolic activity of the treated cells was quantified as a ratio of untreated cells utilized as a negative control.28
Flow cytometry
The impact of PMP, Ara-C, and their respective combinations on initiating early and late apoptosis was assessed through annexin-V and propidium iodide (PI) staining. A total of 4 × 105 cells were seeded per well on a six-well cell culture plate and subjected to treatment with PMP (400 µg/mL), Ara-C (1.5 µM), or a combination of both (400 µg/mL PMP and 1.5 µM Ara-C). Subsequently, flow cytometry analysis was performed using the Annexin-V Apoptosis Detection Kit (Mab Tag, AnxF100PI), and the resulting data were analyzed using FlowJo version 7.6.1 software.29
Gene expression analysis using real-time PCR
Real-time PCR was used to monitor changes in mRNA expression of Bax, Bcl-2, P21, and h-TERT genes. Total RNA was isolated using YTzol Pure RNA (Yekta Tajhiz Azma, YT9066) from untreated (control) and treated cells with 400 µg/mL PMP, 1.5 µM Ara-C, and PMP/Ara-C combination (400 µg/mL PMP and 1.5 µM Ara-C) following a 48-hour treatment period. The purity and concentration of the extracted RNA were determined by analyzing the ratio of light absorption at wavelengths of 260 nm and 280 nm using a NanoDrop 1000 Spectrophotometer. Moreover, the quality of the extracted RNA was assessed by subjecting RNA molecules to electrophoresis on an agarose gel for separation. Afterward, cDNA was synthesized according to the manufacturer's instructions (Thermo Scientific Fermentas, K1622). As described previously, real-time PCR was used to monitor changes in the mRNA expression of the desired genes.28 Table 1 shows the nucleotide sequences of the primers used in real-time RT-PCR.
Table 1.
PCR primer sequences
Gene
|
Sequence 5′–3′
|
Tm
|
nt #
|
GAPDH-FW |
CCAACCGCGAGAAGATGA |
50.3 |
18 |
GAPDH-Rv |
TCCATCACGATGCCAGTG |
50.3 |
18 |
Bax-FW |
AGGATCGAGCAGGGCGAATG |
62.5 |
20 |
Bax-Rv |
TCAGCTTCTTGGTGGACGCA |
60.5 |
20 |
P21-FW |
CCTGTCACTGTCTTGTACCCT |
54.4 |
21 |
P21-Rv |
GCGTTTGGAGTGGTAGAAATCT |
53 |
22 |
Bcl-2-FW |
ATCGCCCTGTGGATGACTGAG |
55.9 |
21 |
Bcl-2-Rv |
CAGCCAGGAGAAATCAAACAGAG |
55.9 |
23 |
h-TERT -FW |
CGGAAGAGTGTCTGGAGCAA |
60 |
20 |
h-TERT -Rv |
GGATGAAGCGGAGTCTGGA |
59.3 |
19 |
Statistical analysis
The statistical analysis for this research was conducted using SPSS26 software to assess the assumptions, while GraphPad Prism 8 software was utilized for generating graphs. A significance level of 5% (alpha error) was set as the threshold for either rejecting or confirming the null hypothesis. All mean comparisons were conducted using two-way tests. Statistical significance was determined with a P value below 0.05. For the analysis of cell survival, metabolic activity, apoptosis, and gene expression data, two-way repeated measures ANOVA was employed in this study. Sample size calculation was performed using G-Power software, considering an alpha error of 5%, a test power of 60%, an effect size of 50%, and accounting for four treatments measured at two different time points. The calculated sample size for each group was 3.
Results
Determination of the size of PMPs
The average size of the isolated PMPs was 200 nm for 90%, as shown in Fig. 1.
Fig. 1.
Determining the average size of microparticles derived from platelets by Malvern Master Sizer 2000. The size dispersion of the separated PMP was evaluated within a span of 200 nm.
Fig. 1.
Determining the average size of microparticles derived from platelets by Malvern Master Sizer 2000. The size dispersion of the separated PMP was evaluated within a span of 200 nm.
Phenotypical characterization of PMPs
The immunophenotypic characterization revealed that 97% of the PMPs expressed CD61 (Fig. 2a), and 95% expressed CD42b (Fig. 2b) on their surface.
Fig. 2.
Flow cytometry analysis revealed a high expression of CD61 (a) molecules (97.6%) and CD42b (b) molecules (95%) on the PMP.
Fig. 2.
Flow cytometry analysis revealed a high expression of CD61 (a) molecules (97.6%) and CD42b (b) molecules (95%) on the PMP.
The impact of Ara-C on the cell mortality of Nalm-6 cells was increased after co-incubation with PMP
The effects of different concentrations of Ara-C (0.5, 1, 1.5, and 2 µM) and PMP (200, 400, and 800 µg/mL), both individually and in combination, on cell viability were studied at 24, 48, and 72 hours. According to Fig. 3a, PMP treatment did not affect the viability of Nalm-6 cells. In contrast, as illustrated in Fig. 3b, the Nalm-6 cell viability decreased following exposure to Ara-C. Notably, co-incubation of cells with the combination of Ara-C and PMP for 48 h of treatment reduced cell viability more effectively than to Ara-C alone, as illustrated in Fig. 3c.
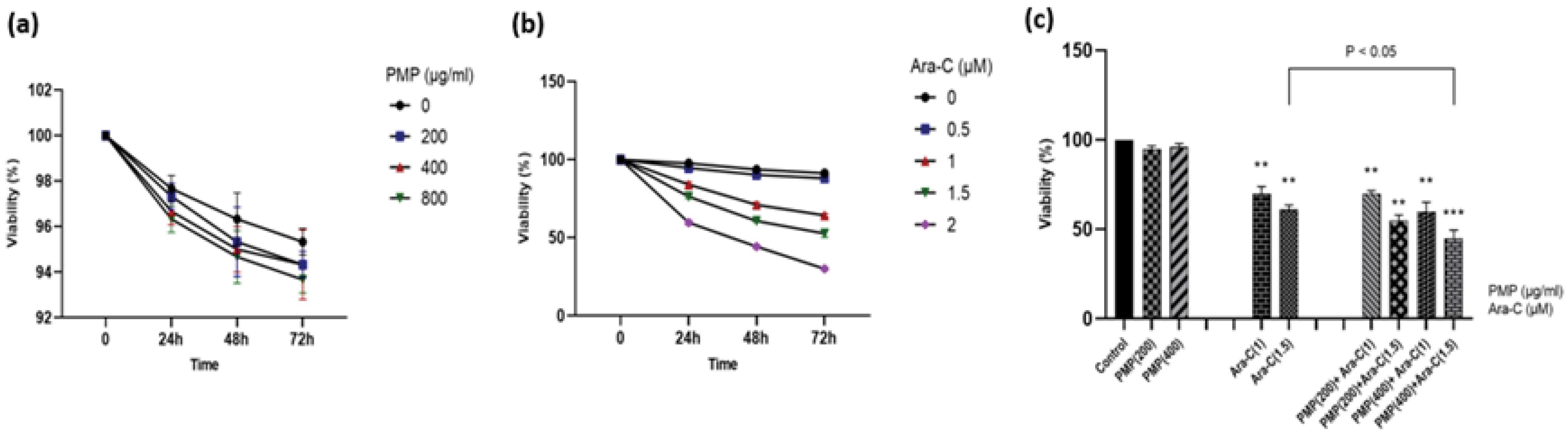
Fig. 3.
The viability of Nalm-6 cells was assessed for 24, 48, and 72 h to determine the impact of different doses of (a) PMP, (b) Ara-C, and (c) the combination of PMP and Ara-C. The assessment was conducted using trypan blue staining, and the results were presented as mean ± standard error (SE) with a sample size of 3. Interestingly, the various concentrations of PMP (200, 400, and 800 µg/mL) did not show any significant effect on Nalm-6 cell viability. However, the effects of Ara-C (0.5, 1, 1.5, and 2 µM) were found to be dose- and time-dependent. This suggests that the viability of Nalm-6 cells was influenced by the concentration of Ara-C and the duration of exposure. Furthermore, the impact of PMP and Ara-C in combination on Nalm-6 cell viability was evaluated after 48 h. The results indicated that the combination dose significantly reduced cell viability compared to Ara-C alone. This suggests a potential synergistic effect between PMP and Ara-C in inhibiting the viability of Nalm-6 cells (**P < 0.01, ***P < 0.001 relative to untreated cells).
Fig. 3.
The viability of Nalm-6 cells was assessed for 24, 48, and 72 h to determine the impact of different doses of (a) PMP, (b) Ara-C, and (c) the combination of PMP and Ara-C. The assessment was conducted using trypan blue staining, and the results were presented as mean ± standard error (SE) with a sample size of 3. Interestingly, the various concentrations of PMP (200, 400, and 800 µg/mL) did not show any significant effect on Nalm-6 cell viability. However, the effects of Ara-C (0.5, 1, 1.5, and 2 µM) were found to be dose- and time-dependent. This suggests that the viability of Nalm-6 cells was influenced by the concentration of Ara-C and the duration of exposure. Furthermore, the impact of PMP and Ara-C in combination on Nalm-6 cell viability was evaluated after 48 h. The results indicated that the combination dose significantly reduced cell viability compared to Ara-C alone. This suggests a potential synergistic effect between PMP and Ara-C in inhibiting the viability of Nalm-6 cells (**P < 0.01, ***P < 0.001 relative to untreated cells).
The cytotoxic impact of Ara-C and PMP on Nalm-6 cells intensified following concurrent incubation
The cytotoxic impact of PMP and Ara-C at different concentrations (1, 1.5 µM for Ara-C; 200, 400, and 800 µg/mL for PMP) and their combination was assessed. PMP and Ara-C demonstrated independent cytotoxic effects on Nalm-6 treated cells (Fig. 4a, b). The concurrent treatment of PMP and Ara-C synergistically amplified the cytotoxic effects on the Nalm-6 cells (Fig. 4c). Synergistic effects (CI < 1) were observed for two combination doses: PMP (400 µg/mL) + Ara-C (1 µM) and PMP (400 µg/mL) + Ara-C (1.5 µM). An additive effect (CI = 1) was noted for PMP (200 µg/mL) + Ara-C (1 µM), and an antagonistic effect (CI > 1) for PMP (200 µg/mL) + Ara-C (1.5 µM) (Fig. 5). Table 2 displays the CI and DRI values for the combination treatments, with the combination of 1.5 μM Ara-C and 400 µg/mL PMP showing strong synergistic effects, chosen for subsequent experiments.
Fig. 4.
The cytotoxic effect of PMP (a) and Ara-C (b) on Nalm-6 cells was conducted at 24, 48, and 72 hours following treatment. Both PMP and Ara-C demonstrated toxicity effects. Additionally, (c) the collective impact of PMP and Ara-C was evaluated in comparison to separate dosages following a 48-hour period. PMP and Ara-C exhibited distinct cytotoxic impacts on Nalm-6 cells. The concurrent administration of PMP and Ara-C demonstrated a significant influence. Significant effects of the drug combination were noted in four specific doses (PMP and Ara-C: 200 + 1, 200 + 1.5, 400 + 1, 400 + 1.5) (*P < 0.05, **P < 0.01, ***P < 0.001, relative to untreated cells).
Fig. 4.
The cytotoxic effect of PMP (a) and Ara-C (b) on Nalm-6 cells was conducted at 24, 48, and 72 hours following treatment. Both PMP and Ara-C demonstrated toxicity effects. Additionally, (c) the collective impact of PMP and Ara-C was evaluated in comparison to separate dosages following a 48-hour period. PMP and Ara-C exhibited distinct cytotoxic impacts on Nalm-6 cells. The concurrent administration of PMP and Ara-C demonstrated a significant influence. Significant effects of the drug combination were noted in four specific doses (PMP and Ara-C: 200 + 1, 200 + 1.5, 400 + 1, 400 + 1.5) (*P < 0.05, **P < 0.01, ***P < 0.001, relative to untreated cells).
Fig. 5.
The synergistic effects of the combined therapy involving PMP and Ara-C on Nalm-6 cells were evaluated through the analysis of the CI versus FA Curve. Different combinations of PMP and Ara-C were administered, and their effect on the metabolic activity of the cells was evaluated using the MTT assay to generate CI vs FA data. (a) CI values below 1 indicate a synergistic effect, values at 1 represent an additive effect (shown by the solid line), and values above 1 indicate antagonism. It is worth noting that the combination doses of PMP (400 µg/mL) + Ara-C (1 µM) and PMP (400 µg/mL) + Ara-C (1.5 µM) exhibited significant synergistic effects. (b) Furthermore, the dose-normalized isobologram analysis demonstrated that points below the effect line indicate synergism, revealing a synergistic effect for two combination points in Nalm-6 cells.
Fig. 5.
The synergistic effects of the combined therapy involving PMP and Ara-C on Nalm-6 cells were evaluated through the analysis of the CI versus FA Curve. Different combinations of PMP and Ara-C were administered, and their effect on the metabolic activity of the cells was evaluated using the MTT assay to generate CI vs FA data. (a) CI values below 1 indicate a synergistic effect, values at 1 represent an additive effect (shown by the solid line), and values above 1 indicate antagonism. It is worth noting that the combination doses of PMP (400 µg/mL) + Ara-C (1 µM) and PMP (400 µg/mL) + Ara-C (1.5 µM) exhibited significant synergistic effects. (b) Furthermore, the dose-normalized isobologram analysis demonstrated that points below the effect line indicate synergism, revealing a synergistic effect for two combination points in Nalm-6 cells.
Table 2.
CI and DRI for drug combination by Ara-C and PMP
Ara-C (µM)
|
DRI
|
PMP
(µg/mL)
|
DRI
|
CI value (At inhibition of 50%)
|
1.0 |
1.5 |
200 |
2.757 |
1.029 |
1.0 |
2.231 |
400 |
1.889 |
0.977 |
1.5 |
1.172 |
200 |
3.129 |
1.172 |
1.5 |
3.32 |
400 |
3.568 |
0.581 |
PMP enhanced Ara-C apoptosis induced by Ara-C
The impact of Ara-C alone and in combination with PMP on programmed cell death was explored. Fig. 6a shows the control group that was not exposed to any treatment. Fig. 6b illustrates that the co-incubation of Nalm-6 cells with PMP for 48 hours did not lead to a notable effect on cell apoptosis. Annexin V + /PI + cells in the Ara-C treated group increased significantly (Fig. 6c). According to Fig. 6d, 48 h co-treatment of Nalm-6 cells with the combination of 1.5μM Ara-C plus 400μg/ml PMP induced cell death more than the Ara-C group. These findings suggest that PMP enhances the apoptotic effect of Ara-C.
Fig. 6.
Nalm-6 cells were subjected to various treatments, including control (untreated) (a), PMP: 400 µg/mL (b), Ara-C: 1.5 µM (c), and PMP: 400/Ara-C: 1.5 (d) for a duration of 48 h. Flow cytometry was utilized to evaluate Annexin-V and Annexin-V plus Propidium Iodide (PI) expression. Following a 48 h co-incubation with PMP, there was no significant impact on cell apoptosis in Nalm-6 cells. Conversely, Annexin V + /PI + cells notably increased in the Ara-C treated group. It is apparent that PMP significantly enhanced the apoptotic effect of Ara-C on Nalm-6 cells (*P < 0.05, **P < 0.01, ***P < 0.001, ****P < 0.0001 relative to untreated cells).
Fig. 6.
Nalm-6 cells were subjected to various treatments, including control (untreated) (a), PMP: 400 µg/mL (b), Ara-C: 1.5 µM (c), and PMP: 400/Ara-C: 1.5 (d) for a duration of 48 h. Flow cytometry was utilized to evaluate Annexin-V and Annexin-V plus Propidium Iodide (PI) expression. Following a 48 h co-incubation with PMP, there was no significant impact on cell apoptosis in Nalm-6 cells. Conversely, Annexin V + /PI + cells notably increased in the Ara-C treated group. It is apparent that PMP significantly enhanced the apoptotic effect of Ara-C on Nalm-6 cells (*P < 0.05, **P < 0.01, ***P < 0.001, ****P < 0.0001 relative to untreated cells).
The impact of the PMP/Ara-C combination on gene expression
The expression of genes associated with cell death was examined further to study the effect of the PMP on Ara-C-dependent apoptosis. Ara-C upregulated the expression of the Bax gene, as illustrated in Fig. 7a, while PMP had no impact on Bax gene expression. The combination of PMP and Ara-C resulted in a higher Bax gene expression compared to Ara-C treatment alone, as indicated by the results. The results (Fig. 7b) revealed that PMP did not affect the Bcl-2 gene in Nalm-6 cells. However, Ara-C decreased its expression. When cells were co-incubated with PMP and Ara-C, the expression of this gene was less than in the control group, Ara-C alone, and PMP alone. These results showed that PMP increases Ara-C-induced apoptosis in Nalm-6 cells. To further understand the effect of the PMP/Ara-C combination on the molecular mechanism of cell population reduction, the expression of the proliferative gene (P21) and the gene associated with telomerase (h-TERT) was studied. As seen in Fig. 7c, P21 gene expression increases in all three groups, although it is more significant in the combined group than in the other two. PMP treatment increased h-TERT gene expression in Nalm-6 cells, but Ara-C treatment decreased h-TERT gene expression. Furthermore, co-treatment of cells with PMP plus Ara-C resulted in a substantial reduction in h-TERT gene expression (Fig. 7d).
Fig. 7.
Fold change gene expression. (a) The graph presents that PMP does not affect Bax gene expression, but Ara-C upregulates the Bax gene (proapoptotic). (b) Also, PMP does not affect Bcl-2 gene expression, but Ara-C downregulates the Bcl-2 gene (anti-apoptotic). After 48 h of treatment, a combination of PMP and Ara-C induces more significant changes in gene expression compared to single doses in Nalm-6 cells. (c) P21 gene expression increases in all three groups, although it is more significant in the combined group than in the other two. (d) Also, PMP treatment increased h-TERT gene expression in Nalm-6 cells, but Ara-C treatment decreased h-TERT gene expression. Furthermore, the combined dosage resulted in a substantial reduction in h-TERT gene expression (*P < 0.05, **P < 0.01, ***P < 0.001, relative to untreated cells).
Fig. 7.
Fold change gene expression. (a) The graph presents that PMP does not affect Bax gene expression, but Ara-C upregulates the Bax gene (proapoptotic). (b) Also, PMP does not affect Bcl-2 gene expression, but Ara-C downregulates the Bcl-2 gene (anti-apoptotic). After 48 h of treatment, a combination of PMP and Ara-C induces more significant changes in gene expression compared to single doses in Nalm-6 cells. (c) P21 gene expression increases in all three groups, although it is more significant in the combined group than in the other two. (d) Also, PMP treatment increased h-TERT gene expression in Nalm-6 cells, but Ara-C treatment decreased h-TERT gene expression. Furthermore, the combined dosage resulted in a substantial reduction in h-TERT gene expression (*P < 0.05, **P < 0.01, ***P < 0.001, relative to untreated cells).
Discussion
Treatment resistance poses a significant challenge for individuals with ALL despite advancements in pharmacological therapies.30 While leukemia treatments have become more effective and less toxic over time, mortality rates persist as a concern. Combined therapy is often recommended to reduce cytotoxic dosages while enhancing treatment efficacy. Ara-C has demonstrated effectiveness in treating various hematologic cancers, including ALL.31 Numerous studies have explored the potential of combination therapy to improve ALL treatment, involving Ara-C and other drugs.32 Various factors within the tumor microenvironment play crucial roles in determining the sensitivity of cells to therapeutic drugs in different types of cancers.33 Platelets contain growth factors, inflammatory cytokines, and immune factors that modify tumor biology.34 The intricate roles of platelet cargo in cancer are still not fully understood, with their influence probably varying based on factors like tumor type and stage.35 PMPs have attracted considerable interest due to their potential involvement in facilitating communication between platelets and cancer cells.36 PMPs influence cancer cell behavior by delivering a varied payload of nucleic acids, proteins, and lipids.37-39 There has been a significant increase in research focusing on PMP and its roles in various cellular functions and disease states.40 Recently, considerable investigation has focused on the impact of PMPs in combination with chemotherapy drugs, mainly through in vitro studies. For example, Cacic et al demonstrated that PMPs protect the THP-1 cell line against daunorubicin-induced apoptosis.41 Additionally, in 2023, Gharib et al studied the resistance of cell-related chronic lymphocytic leukemia cells treated with PMP against cytarabine.42 These findings collectively advance our understanding of the intricate interplay between PMPs and chemotherapy agents in cancer treatment. Despite numerous studies on the interaction of PMPs and cancer, our understanding of their effectiveness in combination with chemotherapy drugs remains largely inadequate and necessitates further comprehensive research. In this research, we explore the cytotoxic and apoptotic effects of PMP, Ara-C, and their combinations on Nalm-6 cells, as well as their influence on the expression of critical genes like Bax, Bcl-2, P21, and h-TERT. Our study confirms the tumor-inhibiting effect of PMPs on cell lines in vitro. The cytotoxic effect of PMP on Nalm-6 cells and reduction in metabolic activity of Nalm-6 cells after PMP treatment is consistent with previous research indicating a potential anti-leukemic influence associated with PMPs. Vismora et al showed that PMPs significantly reduced the metabolic activity of breast cancer cells without notably affecting their viability.44 Additionally have been shown that PMPs inhibit mitochondrial function in the lung and colon cells in mice.45 Our study demonstrates that both PMP and Ara-C exhibit cytotoxicity and reduce metabolic activity in treated Nalm-6 cells. Moreover, the concurrent treatment with PMP and Ara-C synergistically enhances the cytotoxic effects on cells. Studies have shown varying results regarding the impact of PMPs on cancer metabolism. A study examined the influence of PMPs on cell lines associated with chronic lymphocytic leukemia, revealing a significant metabolic change from early to later stages.42 Furthermore, the CompuSyn analysis demonstrated significant synergistic effects on Nalm-6 cells with two combination doses of Ara-C and PMP (400 µg/mL + 1 µM and 400 µg/mL + 1.5 µM). These findings highlight the potential synergistic impact of PMP and Ara-C combination therapy on Nalm-6 cells, suggesting a promising avenue for further investigation. CD36, GNAI2, GNB2, PPP2R1A, PRKCA, PRKACB, PPP2CA, PRKAR1A, ITPR1, FASN, GNAQ, GNG5, and TKT are among the proteins associated with PMPs that play crucial roles in diverse signaling pathways and regulate the metabolic activity of target cells.46 Additionally, another study suggests that PMPs may influence metabolic activity by transferring genetic materials, such as miRNAs.45
Co-incubation of Nalm-6 cells with PMPs increased their sensitivity to Ara-C at a concentration of 1.5 μM Ara-C plus 400 μg/ml PMP. Studies have shown PMPs potentially influence cellular signaling pathways that sensitize cells to apoptosis. Baj-Krzyworzeka and colleagues conducted a study examining the effects of PMPs on hematological cell lines, revealing that PMPs activate MAPKp42/44 and PI-3K-AKT in hematopoietic cells.47 Moreover, Fateme Yari et al. showed that PMP induces apoptosis in PBMCs isolated from pre-B acute lymphoblastic leukemia.43 Several studies have observed that PMPs affect cancer cell apoptosis induced by chemotherapy drugs. For instance, Cacic and colleagues demonstrated that co-incubation of THP-1 cells with PMPs elevated levels of miR-125a, miR-125b, and miR-199, which protected THP-1 cells against daunorubicin-induced cell death.48 Recent findings also highlight the role of PMPs in the metabolic reprogramming of CLL cells, contributing to their increased resistance to chemotherapy drugs such as cytarabine, venetoclax, and plumbagin.42
To further investigate the underlying mechanisms, we assessed the impact of PMPs, Ara-C, and their combination on the expression of apoptotic genes like Bax and Bcl-2. Bcl-2 and Bcl-XL, members of the anti-apoptotic Bcl-2 family, impede cell death by inhibiting caspase activation crucial for apoptosis or by preventing the release of apoptotic factors like cytochrome C and AIF from the mitochondria.49 In this study, the expression of the Bcl-2 gene in cells treated with PMPs did not show significant differences compared to the control group. However, gene expression decreased in cells exposed to Ara-C alone and in combination with PMPs. Importantly, PMPs did not affect the expression of the Bax gene. Nevertheless, Bax gene expression was higher in cells treated with both PMPs and Ara-C compared to cells treated with Ara-C alone. The analysis of Bax and Bcl-2 gene expressions provides a plausible explanation for the increased susceptibility of Nalm-6 cells to chemotherapy drugs following treatment with PMPs and Ara-C.
Previous research has shown that PMPs transfer their genetic material, including miRNAs, to target cells, influencing gene expression and the apoptotic response in laboratory settings.50 PMPs contain a rich reservoir of proteins, lipids, second messengers, and various RNA species. These components are transferred to target cells, altering cell signaling pathways and modulating the cellular response to drugs.51 However, the exact mechanisms underlying these changes in drug response are not fully understood and require further investigation.
We also examined the effects of PMP, Ara-C, and the combination of these two on the expression of P21 and h-TERT genes. The study findings revealed that PMP treatment led to an increase in the expression of the P21 gene, with molecular data consistently aligning with the observed decrease in cell proliferation in cell culture. The results revealed that treating Nalm-6 cells with Ara-C, either alone or in combination with PMPs, enhanced the expression of the P21 gene. The influence of PMPs on proliferation control and cell population was assessed using Neobar slides and a cell counter. The results showed a significant decrease in cell population after treatment with both PMPs and Ara-C. The inhibitory effect of Ara-C was further enhanced when combined with PMP treatment.
Several studies have highlighted the potential tumor-suppressing properties of PMPs, demonstrating their ability to inhibit tumor progression in mouse models of lung and colon cancer.45 Furthermore, in laboratory experiments, PMPs have been observed to suppress the growth of cancer cell lines, such as THP-1 and HUVEC.37,41 Contrary to the findings in the study conducted by Mirzaee et al, it was demonstrated that PMPs reduce the expression of P21 and P53 genes in Mesenchymal Stem Cells, leading to increased cell proliferation. The differing effects observed in different cell lines suggest that the impact of PMPs may vary depending on the specific type of target cell.23
The P21 gene inhibits cancer cell proliferation through pathways that involve both P53-dependent and independent mechanisms. These pathways include regulating metabolic pathways and triggering cellular senescence.52 Ensuring the longevity of telomeres through the activation of telomerase, particularly h-TERT, is essential for the continuous renewal of cells, which is a hallmark of cancer. The regulation of h-TERT transcription is critical for activating telomerase in human cancers. This underscores the importance of mechanisms that govern telomere maintenance in cancer development and their potential as indicators in oncology.53 While telomerase activity is typically observed in germline, hematopoietic, and rapidly renewing cells, this study sheds light on the intriguing interplay between PMP and h-TERT gene expression, demonstrating that PMP can provoke a notable increase in h-TERT gene expression levels. In the investigation by Samareh et al, real-time PCR analysis revealed a remarkable over threefold surge in h-TERT gene expression upon treatment with PMP, compared to the control group.53 Ara-C appears to cause a reduction in the expression of this gene. Interestingly, when Ara-C is given with PMP, it lessens the inhibitory effect of Ara-C on h-TERT gene expression. However, the precise mechanism behind this observed phenomenon is still unclear and requires additional investigation. Our study underscores the influence of PMPs on cancer biology. PMPs reduce cell proliferation and have a cytotoxic effect on cells, impacting gene expression both alone and in on with Ara-C. These results underscore the complex relationship between PMPs and cancer cell behavior, providing valuable insights for enhancing therapeutic strategies in cancer treatment.
Furthermore, our study is consistent with previous investigations,54 contrasting with reports of PMPs enhancing cancer progression in other studies.15,47 This dual potential of PMPs to either promote cancer progression or suppress tumor growth suggests a complex interaction between cancer cells and PMPs, likely influenced by factors such as the conditions of PMP creation, cell type, and cancer stage.
It is important to clarify that this study specifically investigated the interaction between PMPs and Nalm-6 cells, as well as the combined effect of PMPs and Ara-C, in an in vitro setting.
It is worth noting that results obtained in vivo may vary due to the influence of environmental factors and the interplay between the cell line and the immune system. Moreover, the specific contents of PMPs and their mechanism of action remain crucial considerations for understanding their effects, However, these aspects were not investigated in our study, which aimed to explore the overall impact of PMPs.
To strengthen the validity of our findings, future experiments should confirm our results using a wider variety of leukemia cell lines and additional chemotherapy drugs. This broader approach will help ensure the reliability and applicability of our conclusions across different contexts and treatments in leukemia research. Additionally, including samples from patients in the early stages of leukemia would provide valuable insights into the potential applicability of PMPs in diverse clinical contexts. This comprehensive approach is essential for gaining a deeper understanding of the therapeutic potential of PMPs in cancer treatment.
Advancing our understanding of the dynamic relationship between cancer cells and PMPs holds significant implications for potential therapeutic interventions. However, additional research is needed to uncover the precise mechanisms underlying PMPs' role in modulating cancer cell behavior. Specifically, efforts should focus on elucidating the specific molecules responsible for these effects.
Conclusion
Our study offers a new point of perspective on the interaction between PMPs and cancer, indicating that PMPs have the potential to impact cancer cell behavior and trigger unique reactions. These findings reinforce the expanding evidence on the anti-tumor properties of PMPs and emphasize the necessity for comprehensive investigations to grasp their underlying mechanisms fully. Our research underscores the significance of examining the influence of environmental factors like PMPs in cancer biology, which could ultimately pave the way for developing more efficacious treatments for leukemia and various other cancer types.
Research Highlights
What is the current knowledge?
What is new here?
-
PMPs enhance Ara-C-induced apoptosis in Nalm-6 cells.
-
PMPs modulate the expression of critical genes Bax, Bcl-2, P21, and h-TERT.
Competing Interests
The authors declare that they have no conflict of interest.
Ethical Statement
This study was performed in line with the principles of the Declaration of Helsinki. Approval was granted by the Ethics Committee of the Vice Chancellor for Research and Technology of Kerman University of Medical Sciences (IR.KMU.REC.1399.420). Informed consent was obtained from all individual participants included in the study.
Acknowledgements
We sincerely thank the Kerman Blood Transfusion Center.
References
- Cao Y, Wu C, Song Y, Lin Z, Kang Y, Lu P. Cyr61 decreases cytarabine chemosensitivity in acute lymphoblastic leukemia cells via NF-κB pathway activation. Int J Mol Med 2019; 43:1011-20. doi: 10.3892/ijmm.2018.4018 [Crossref] [ Google Scholar]
- Jabbour E, O'Brien S, Konopleva M, Kantarjian H. New insights into the pathophysiology and therapy of adult acute lymphoblastic leukemia. Cancer 2015; 121:2517-28. doi: 10.1002/cncr.29383 [Crossref] [ Google Scholar]
- Mei L, Ontiveros EP, Griffiths EA, Thompson JE, Wang ES, Wetzler M. Pharmacogenetics predictive of response and toxicity in acute lymphoblastic leukemia therapy. Blood Rev 2015; 29:243-9. doi: 10.1016/j.blre.2015.01.001 [Crossref] [ Google Scholar]
- Inaba H, Mullighan CG. Pediatric acute lymphoblastic leukemia. Haematologica 2020; 105:2524-39. doi: 10.3324/haematol.2020.247031 [Crossref] [ Google Scholar]
- Terwilliger T, Abdul-Hay M. Acute lymphoblastic leukemia: a comprehensive review and 2017 update. Blood Cancer J 2017; 7:e577. doi: 10.1038/bcj.2017.53 [Crossref] [ Google Scholar]
- Li Z, Guo JR, Chen QQ, Wang CY, Zhang WJ, Yao MC. Exploring the antitumor mechanism of high-dose cytarabine through the metabolic perturbations of ribonucleotide and deoxyribonucleotide in human promyelocytic leukemia HL-60 cells. Molecules 2017; 22:499. doi: 10.3390/molecules22030499 [Crossref] [ Google Scholar]
- Reese ND, Schiller GJ. High-dose cytarabine (HD araC) in the treatment of leukemias: a review. CurrHematolMalig Rep 2013; 8:141-8. doi: 10.1007/s11899-013-0156-3 [Crossref] [ Google Scholar]
- Di Francia R, Crisci S, De Monaco A, Cafiero C, Re A, Iaccarino G. Response and toxicity to cytarabine therapy in leukemia and lymphoma: from dose puzzle to pharmacogenomic biomarkers. Cancers (Basel) 2021; 13:966. doi: 10.3390/cancers13050966 [Crossref] [ Google Scholar]
- Lato MW, Przysucha A, Grosman S, Zawitkowska J, Lejman M. The new therapeutic strategies in pediatric T-cell acute lymphoblastic leukemia. Int J Mol Sci 2021; 22:4502. doi: 10.3390/ijms22094502 [Crossref] [ Google Scholar]
- Repsold L, Pool R, Karodia M, Tintinger G, Joubert AM. An overview of the role of platelets in angiogenesis, apoptosis and autophagy in chronic myeloid leukaemia. Cancer Cell Int 2017; 17:89. doi: 10.1186/s12935-017-0460-4 [Crossref] [ Google Scholar]
- Lazar S, Goldfinger LE. Platelet microparticles and miRNA transfer in cancer progression: many targets, modes of action, and effects across cancer stages. Front Cardiovasc Med 2018; 5:13. doi: 10.3389/fcvm.2018.00013 [Crossref] [ Google Scholar]
- Boilard E. Platelet-derived nanomedicine targets cancer. Blood 2017; 130:561-2. doi: 10.1182/blood-2017-05-786327 [Crossref] [ Google Scholar]
- Saber SH, Ali HE, Gaballa R, Gaballah M, Ali HI, Zerfaoui M. Exosomes are the driving force in preparing the soil for the metastatic seeds: lessons from the prostate cancer. Cells 2020; 9:564. doi: 10.3390/cells9030564 [Crossref] [ Google Scholar]
- Evangelista FC, Ferrão AL, Duarte RC, Gomes LC, Alves LC, Campos FM. Circulating microparticles and thrombin generation in patients with chronic lymphocytic leukemia. Braz J Pharm Sci 2022; 58:e19407. doi: 10.1590/s2175-97902022e19407 [Crossref] [ Google Scholar]
- Liang H, Yan X, Pan Y, Wang Y, Wang N, Li L. MicroRNA-223 delivered by platelet-derived microvesicles promotes lung cancer cell invasion via targeting tumor suppressor EPB41L3. Mol Cancer 2015; 14:58. doi: 10.1186/s12943-015-0327-z [Crossref] [ Google Scholar]
- Noulsri E. Effects of cell-derived microparticles on immune cells and potential implications in clinical medicine. Lab Med 2021; 52:122-35. doi: 10.1093/labmed/lmaa043 [Crossref] [ Google Scholar]
- Anderson NM, Harrold I, Mansour MR, Sanda T, McKeown M, Nagykary N. BCL2-specific inhibitor ABT-199 synergizes strongly with cytarabine against the early immature LOUCY cell line but not more-differentiated T-ALL cell lines. Leukemia 2014; 28:1145-8. doi: 10.1038/leu.2013.377 [Crossref] [ Google Scholar]
- Heo SK, Noh EK, Yu HM, Kim DK, Seo HJ, Lee YJ. Radotinib enhances cytarabine (Ara-C)-induced acute myeloid leukemia cell death. BMC Cancer 2020; 20:1193. doi: 10.1186/s12885-020-07701-8 [Crossref] [ Google Scholar]
- Wu PS, Wang CY, Hsu HJ, Yen JH, Wu MJ. 8-Hydroxydaidzein induces apoptosis and inhibits AML-associated gene expression in U-937 cells: potential phytochemical for AML treatment. Biomolecules 2023; 13:1575. doi: 10.3390/biom13111575 [Crossref] [ Google Scholar]
- Zhou Y, Yu F, Luo B, Luo H, Liu C. Cytrarabine (Ara-c) promotes cell apoptosis by inhibiting the phosphorylation of protein kinase B (AKT/PKB). Process Biochem 2019; 82:144-52. doi: 10.1016/j.procbio.2019.04.007 [Crossref] [ Google Scholar]
- Samareh Salavatipour M, Hoseinpour Kasgari F, Farsinejad A, Fatemi A, Mirzaee Khalilabadi R. Platelet-derived microparticles increase expression of hTERT in umbilical cord mesenchymal stem cells. Res Mol Med 2018; 5:31-40. doi: 10.18502/rmm.v5i4.3063 [Crossref] [ Google Scholar]
- Labrie A, Marshall A, Bedi H, Maurer-Spurej E. Characterization of platelet concentrates using dynamic light scattering. Transfus Med Hemother 2013; 40:93-100. doi: 10.1159/000350362 [Crossref] [ Google Scholar]
- Samareh Salavatipour M, Hoseinpour Kasgari F, Farsinejad A, Fatemi A, Hassanshahi G, Mirzaee Khalilabadi R. Platelet microparticles accelerate proliferation and growth of mesenchymal stem cells through longevity-related genes. Arch Iran Med 2021; 24:607-14. doi: 10.34172/aim.2021.86 [Crossref] [ Google Scholar]
- Aatonen MT, Ohman T, Nyman TA, Laitinen S, Grönholm M, Siljander PR. Isolation and characterization of platelet-derived extracellular vesicles. J Extracell Vesicles 2014; 3:24692. doi: 10.3402/jev.v3.24692 [Crossref] [ Google Scholar]
- Fatemi A, Kazemi A, Kashiri M, Safa M. Elevation of cAMP levels inhibits doxorubicin-induced apoptosis in pre-B ALL Nalm-6 cells through induction of BAD phosphorylation and inhibition of P53 accumulation. Int J Mol Cell Med 2015; 4:94-102. [ Google Scholar]
- Chou T, Martin N. PC Software and User’s Guide: A Computer Program for Quantitation of Synergism and Antagonism in Drug Combinations, and the Determination of IC50, ED50 and LD50 Values. Paramus, NJ: ComboSyn; 2005.
- Anani H, Baluchi I, Farsinejad A, Fatemi A, Mirzaee khalilabadi R. Betulinic acid exerts antitumor effects on acute promyelocytic leukemia cells possibly via hTERT downregulation. Gene Rep 2022; 26:101435. doi: 10.1016/j.genrep.2021.101435 [Crossref] [ Google Scholar]
- Anani H, Baluchi I, Farsinejad A, Fatemi A, Mirzaee Khalilabadi R. Zataria multiflora methanolic extract has antitumor properties on U266 multiple myeloma cell line. Gene Rep 2020; 20:100655. doi: 10.1016/j.genrep.2020.100655 [Crossref] [ Google Scholar]
- Lashkari M, Fatemi A, Mardani Valandani H, Mirzaee Khalilabadi R. Promising anti-leukemic effect of Zataria multiflora extract in combination with doxorubicin to combat acute lymphoblastic leukemia cells (Nalm-6) (in vitro and in silico). Sci Rep 2022; 12:12657. doi: 10.1038/s41598-022-16943-4 [Crossref] [ Google Scholar]
- Piktel D, Moore JC, Nesbit S, Sprowls SA, Craig MD, Rellick SL. Chemotherapeutic activity of pitavastatin in vincristine resistant B-cell acute lymphoblastic leukemia. Cancers (Basel) 2023; 15:707. doi: 10.3390/cancers15030707 [Crossref] [ Google Scholar]
- Kanno S, Higurashi A, Watanabe Y, Shouji A, Asou K, Ishikawa M. Susceptibility to cytosine arabinoside (Ara-C)-induced cytotoxicity in human leukemia cell lines. Toxicol Lett 2004; 152:149-58. doi: 10.1016/j.toxlet.2004.04.014 [Crossref] [ Google Scholar]
- Chen Y, Gan D, Huang Q, Luo X, Lin D, Hu J. Emodin and its combination with cytarabine induce apoptosis in resistant acute myeloid leukemia cells in vitro and in vivo. Cell PhysiolBiochem 2018; 48:2061-73. doi: 10.1159/000492544 [Crossref] [ Google Scholar]
- Buergy D, Wenz F, Groden C, Brockmann MA. Tumor-platelet interaction in solid tumors. Int J Cancer 2012; 130:2747-60. doi: 10.1002/ijc.27441 [Crossref] [ Google Scholar]
- D'Alessandro R, Messa C, Refolo MG, Carr BI. Modulation of sensitivity and resistance to multikinase inhibitors by microenvironmental platelet factors in HCC. Expert OpinPharmacother 2015; 16:2773-80. doi: 10.1517/14656566.2015.1101065 [Crossref] [ Google Scholar]
- Huong PT, Nguyen LT, Nguyen XB, Lee SK, Bach DH. The role of platelets in the tumor-microenvironment and the drug resistance of cancer cells. Cancers (Basel) 2019; 11:240. doi: 10.3390/cancers11020240 [Crossref] [ Google Scholar]
- Pan Y, Wang Y, Wang Y, Xu S, Jiang F, Han Y. Platelet-derived microvesicles (PMVs) in cancer progression and clinical applications. Clin Transl Oncol 2023; 25:873-81. doi: 10.1007/s12094-022-03014-3 [Crossref] [ Google Scholar]
- Pan Y, Liang H, Liu H, Li D, Chen X, Li L. Platelet-secreted microRNA-223 promotes endothelial cell apoptosis induced by advanced glycation end products via targeting the insulin-like growth factor 1 receptor. J Immunol 2014; 192:437-46. doi: 10.4049/jimmunol.1301790 [Crossref] [ Google Scholar]
- Burnouf T, Chou ML, Lundy DJ, Chuang EY, Tseng CL, Goubran H. Expanding applications of allogeneic platelets, platelet lysates, and platelet extracellular vesicles in cell therapy, regenerative medicine, and targeted drug delivery. J Biomed Sci 2023; 30:79. doi: 10.1186/s12929-023-00972-w [Crossref] [ Google Scholar]
- Indana HA, Puspitawati I, Mayasari DS, Hartopo AB. Association of acute hyperglycemia and diabetes mellitus with platelet-derived microparticle (PDMP) levels during acute myocardial infarction. J ASEAN Fed Endocr Soc 2023; 38:35-40. doi: 10.15605/jafes.038.02.03 [Crossref] [ Google Scholar]
- Ashoub MH, Samareh Salavatipour M, Hoseinpour Kasgari F, Mardani Valandani H, Mirzaee Khalilabadi R. Extracellular microvesicles: biologic properties, biogenesis, and applications in leukemia. Mol Cell Biochem 2024; 479:419-30. doi: 10.1007/s11010-023-04734-y [Crossref] [ Google Scholar]
- Cacic D, Reikvam H, Nordgård O, Meyer P, Hervig T. Platelet microparticles protect acute myelogenous leukemia cells against daunorubicin-induced apoptosis. Cancers (Basel) 2021; 13:1870. doi: 10.3390/cancers13081870 [Crossref] [ Google Scholar]
- Gharib E, Veilleux V, Boudreau LH, Pichaud N, Robichaud GA. Platelet-derived microparticles provoke chronic lymphocytic leukemia malignancy through metabolic reprogramming. Front Immunol 2023; 14:1207631. doi: 10.3389/fimmu.2023.1207631 [Crossref] [ Google Scholar]
- Yaftian M, Yari F, Ghasemzadeh M, Fallah Azad V, Haghighi M. Induction of apoptosis in cancer cells of pre-B ALL patients after exposure to platelets, platelet-derived microparticles and soluble CD40 ligand. Cell J 2018; 20:120-6. doi: 10.22074/cellj.2018.5032 [Crossref] [ Google Scholar]
- Vismara M, Zarà M, Negri S, Canino J, Canobbio I, Barbieri SS. Platelet-derived extracellular vesicles regulate cell cycle progression and cell migration in breast cancer cells. Biochim Biophys Acta Mol Cell Res 2021; 1868:118886. doi: 10.1016/j.bbamcr.2020.118886 [Crossref] [ Google Scholar]
- Michael JV, Wurtzel JGT, Mao GF, Rao AK, Kolpakov MA, Sabri A. Platelet microparticles infiltrating solid tumors transfer miRNAs that suppress tumor growth. Blood 2017; 130:567-80. doi: 10.1182/blood-2016-11-751099 [Crossref] [ Google Scholar]
- Capriotti AL, Caruso G, Cavaliere C, Piovesana S, Samperi R, Laganà A. Proteomic characterization of human platelet-derived microparticles. Anal Chim Acta 2013; 776:57-63. doi: 10.1016/j.aca.2013.03.023 [Crossref] [ Google Scholar]
- Baj-Krzyworzeka M, Majka M, Pratico D, Ratajczak J, Vilaire G, Kijowski J. Platelet-derived microparticles stimulate proliferation, survival, adhesion, and chemotaxis of hematopoietic cells. Exp Hematol 2002; 30:450-9. doi: 10.1016/s0301-472x(02)00791-9 [Crossref] [ Google Scholar]
- Cacic D, Reikvam H, Nordgård O, Meyer P, Hervig T. Platelet microparticles protect acute myelogenous leukemia cells against daunorubicin-induced apoptosis. Cancers (Basel) 2021; 13:1870. doi: 10.3390/cancers13081870 [Crossref] [ Google Scholar]
- Czabotar PE, Garcia-Saez AJ. Mechanisms of BCL-2 family proteins in mitochondrial apoptosis. Nat Rev Mol Cell Biol 2023; 24:732-48. doi: 10.1038/s41580-023-00629-4 [Crossref] [ Google Scholar]
- Velez J, Enciso LJ, Suarez M, Fiegl M, Grismaldo A, López C. Platelets promote mitochondrial uncoupling and resistance to apoptosis in leukemia cells: a novel paradigm for the bone marrow microenvironment. Cancer Microenviron 2014; 7:79-90. doi: 10.1007/s12307-014-0149-3 [Crossref] [ Google Scholar]
- Boilard E, Duchez AC, Brisson A. The diversity of platelet microparticles. CurrOpinHematol 2015; 22:437-44. doi: 10.1097/moh.0000000000000166 [Crossref] [ Google Scholar]
- Xiao BD, Zhao YJ, Jia XY, Wu J, Wang YG, Huang F. Multifaceted p21 in carcinogenesis, stemness of tumor and tumor therapy. World J Stem Cells 2020; 12:481-7. doi: 10.4252/wjsc.v12.i6.481 [Crossref] [ Google Scholar]
- Leão R, Apolónio JD, Lee D, Figueiredo A, Tabori U, Castelo-Branco P. Mechanisms of human telomerase reverse transcriptase (hTERT) regulation: clinical impacts in cancer. J Biomed Sci 2018; 25:22. doi: 10.1186/s12929-018-0422-8 [Crossref] [ Google Scholar]
- Nazari M, Javandoost E, Talebi M, Movassaghpour A, Soleimani M. Platelet microparticle controversial role in cancer. Adv Pharm Bull 2021; 11:39-55. doi: 10.34172/apb.2021.005 [Crossref] [ Google Scholar]